Menu
- Preamble
- Introduction
- Topic 1: Virology Applicable to Vaccine Development
- Topic 2: Immunology and Immune Correlates of Protection
- Topic 3: Vaccinology
- Topic 4: Animal and Human Infection Models for Coronavirus Vaccine Research
- Topic 5: Policy and Financing
- References
- Appendix A: Acronyms
- Appendix B: Contributors
- Appendix C: CVR High-Priority Milestones by Topic Area and Strategic Goal
Executive Summary
The historic, ongoing toll of COVID-19 on health and economic stability worldwide highlights the critical need to make R&D for broadly protective coronavirus vaccines a global priority. SARS-CoV-2, which emerged in 2019 to cause the COVID-19 pandemic, was the third coronavirus in just the past two decades to have emerged from an animal reservoir to cause human epidemics. Severe acute respiratory syndrome coronavirus (SARS-CoV) emerged in 2003, followed by Middle East respiratory syndrome coronavirus (MERS-CoV) in 2012.
Coronaviruses can be highly lethal. MERS-CoV has a 35% case-fatality ratio (CFR), meaning that about one third of infections result in death. For SARS-CoV, approximately 1 in 10 infections result in death (10% CFR). Fortunately, these two coronaviruses do not spread efficiently from person to person. Although SARS-CoV-2 has a much lower CFR, the virus is highly transmissible and has spread rapidly worldwide, resulting in far more deaths. By the end of 2022, SARS-CoV-2 infections had caused more than 650 million confirmed COVID-19 cases and more than 6.6 million deaths.
Beyond the global scourge of the current COVID-19 pandemic, even more concerning is the threat of a new coronavirus in the future that could be both highly transmissible and highly lethal. Thousands of different coronaviruses are circulating in animals worldwide, particularly in bats but also in other mammals and in birds. The trends of the past 20 years are intensifying, with increasing risk of coronaviruses spilling over from animal reservoirs to people, fueled by the rapid expansion of human populations into animal habitats and an increasingly interconnected world.
The limited durability and immunologic protection of currently available SARS-CoV-2 vaccines further highlight the crucial need for a new, proactive approach to develop coronavirus vaccines that provide better and longer protection against both circulating and future SARS-CoV-2 variants and other coronaviruses that have not yet emerged. Currently available COVID-19 vaccines have proven to be safe and effective for the prevention of severe disease and death, and form the backbone of the global public health pandemic response. However, these vaccines do not provide sufficient protection against infection, transmission, and the relentless emergence of new variants that evade the immune response.
The Center for Infectious Disease Research and Policy (CIDRAP) at the University of Minnesota, with funding from the Bill & Melinda Gates Foundation and The Rockefeller Foundation, created this research and development (R&D) roadmap for broadly protective coronavirus vaccines (referred to as the Coronavirus Vaccines Roadmap [CVR]) to serve as a strategic planning tool to facilitate R&D, coordinate funding, and promote stakeholder engagement. The ultimate goal of the CVR is to generate broadly protective vaccines against species and strains of the Coronaviridae virus family.
Primary audiences for this roadmap include academic basic and translational scientists, clinical researchers, funders, public health policymakers, government officials, industry scientists, business leaders, regulators, and advocacy specialists.
Rationale
Over the past two decades, three novel and significantly pathogenic coronaviruses have emerged from animal reservoirs to cause human epidemics or pandemics. Severe acute respiratory syndrome coronavirus (SARS-CoV) emerged in 2003, followed by Middle East respiratory syndrome coronavirus (MERS-CoV) in 2012 and severe acute respiratory syndrome coronavirus-2 (SARS-CoV-2) in 2019. Coronaviruses can be highly lethal to humans, as illustrated by the 35% case-fatality ratio (CFR) for MERS-CoV and the 10% CFR for SARS-CoV. Fortunately, neither MERS-CoV nor SARS-CoV have been shown to spread efficiently between humans. SARS-CoV-2 has a much lower CFR, but because of its high transmissibility, by the end of 2022 had led to more than 650 million reported COVID-19 cases and more than 6.6 million deaths worldwide (WHO 2022a).
The emergence of future coronaviruses that are both highly pathogenic and highly transmissible represents a real and present threat that underscores the critical need for a coordinated R&D initiative to develop broadly protective coronavirus vaccines.
Additionally, the limited durability and immunologic protection (including limited protection against infection) conferred by available SARS-CoV-2 vaccines and natural infection, and the continuing emergence of new viral variants, further highlight the crucial need for a new, proactive approach to develop vaccines that provide greater durability and target continually emerging variants.
Advancing a global R&D agenda for broadly protective coronavirus vaccines is a large, complex endeavor that requires ongoing investment, communication, and coordination among researchers; representatives from governments, industry, multilateral, and nongovernmental organizations; regulators; and public health policymakers. This roadmap provides a framework and timeline to align the coordination, leadership, and investment necessary to achieve these ambitious goals.
A critical overarching goal of R&D efforts for broadly protective coronavirus vaccines is to develop vaccines that are available and appropriate for use worldwide. The speed of bringing initial SARS-CoV-2 vaccines to market was a spectacular accomplishment; however, multiple factors resulted in gross inequities in access to vaccines in remote and low-resource settings. Disparities were fueled by products whose cold-chain and technical requirements limited their use, protection of national interests in the face of limited supply, and global inequities in technical and public health capacity, financing, technology transfer, and manufacturing capabilities.
Future vaccine development must ensure that global equity is a core principle of R&D, and that programs anticipate and resolve issues that may undermine this objective.
Going forward, early and continuous engagement at the community, national, regional, and international levels will be essential to accomplish equitable distribution and uptake of future coronavirus vaccines.
Roadmap scope and structure
Recent efforts to develop R&D roadmaps in other fields, such as medical countermeasure development for World Health Organization (WHO) priority diseases (WHO R&D Blueprint 2022, Modjarrad 2016) and the Influenza Vaccines R&D Roadmap (CIDRAP 2021) informed the structure of the CVR, which is organized into five topic areas:
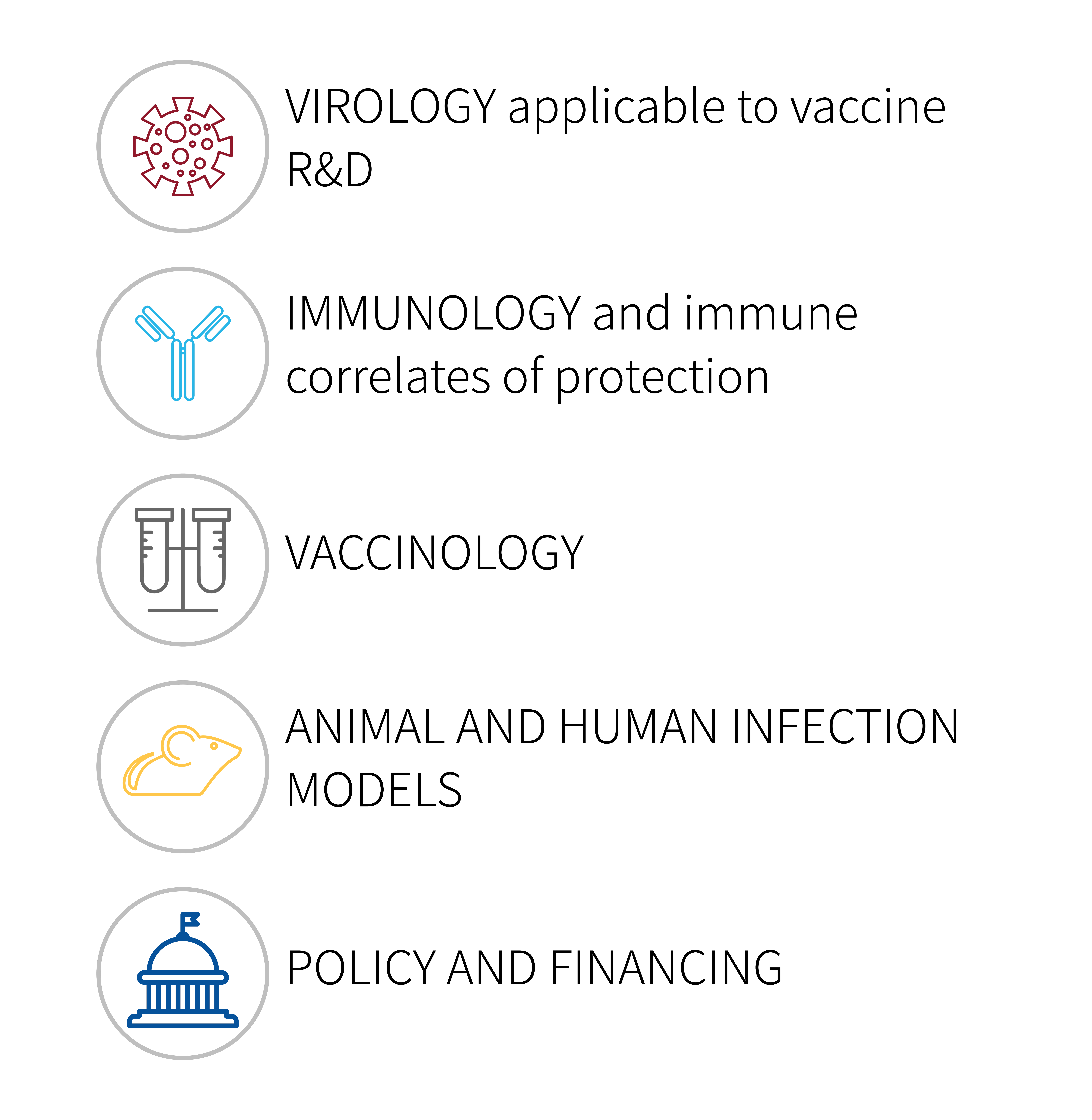
Each topic area contains an overview of key issues, barriers, and knowledge gaps germane to that section. Building on those issues, high-level strategic goals within the five topic areas are identified, followed by associated actions (milestones) required to achieve them. The milestones include target dates for completion (by end of the year listed for each milestone) and reflect SMART (specific, measurable, achievable, realistic/relevant, and time-sensitive) criteria, to the degree feasible. High-priority milestones are those that are most critical toward developing new, broadly protective coronavirus vaccines. In some instances, milestones are aspirational, in that they reflect an important area of research and include somewhat optimistic timelines to help move the area forward. Each topic area also includes a list of additional research priorities. These lists are not meant to be comprehensive and may be subject to reprioritization based on future scientific discovery, but rather are intended to illustrate additional areas of interest for future research. Items listed under this heading generally fall into one of the following categories: (1) the item is not of high enough priority to be included in the goals and milestones, (2) the nature of the research or activity does not lend itself to an initial target date for completion (recognizing that research for many of the milestones in the CVR will continue to be further refined over time even after the initial target is met), or (3) the research or activity is relatively nonspecific and not amenable to milestone criteria.
This roadmap takes a holistic view of the myriad complex scientific and policy issues that are important for generating broadly protective coronavirus vaccines that are suitable for use in all countries and will protect against existing coronaviruses known to cause serious disease in humans (including new SARS-CoV-2 variants of concern) and any other pre-emergent coronaviruses that could spill over from zoonotic reservoirs to humans in the future. However, we also recognize that some of the issues identified in this roadmap are aimed at moving this field of research forward in the long term and are not necessarily essential for early successful development of next-generation vaccines. In the short term, therefore, exploring less resource-intensive, targeted vaccinology solutions may offer a more pragmatic approach toward early development of more broadly protective coronavirus vaccines.
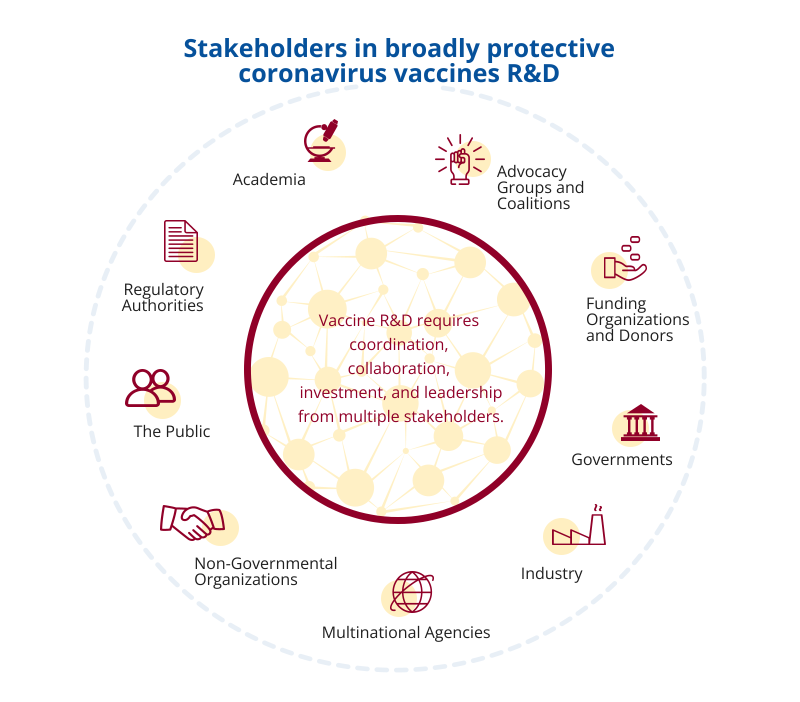
The CVR focuses specifically on issues related to an R&D agenda; a number of issues, although critical to vaccine distribution and uptake, are beyond the scope of this roadmap. Examples include policy and practices related to current SARS-CoV-2 vaccines (such as dosing schedules and frequency of boosters); vaccine hesitancy issues; general public health prevention and control measures; and implementation of national or local vaccine distribution programs.
Roadmap development process
The CVR development process engaged a wide range of stakeholders across scientific disciplines, public and private sectors, and international communities to build consensus around R&D priorities and identify strategies for addressing them. The process included identifying and reviewing relevant scientific literature, discussing scientific challenges and knowledge gaps with a range of subject-matter experts (SMEs) from different fields, conducting in-depth reviews of draft roadmap documents, and offering a widely publicized public comment period for written feedback.
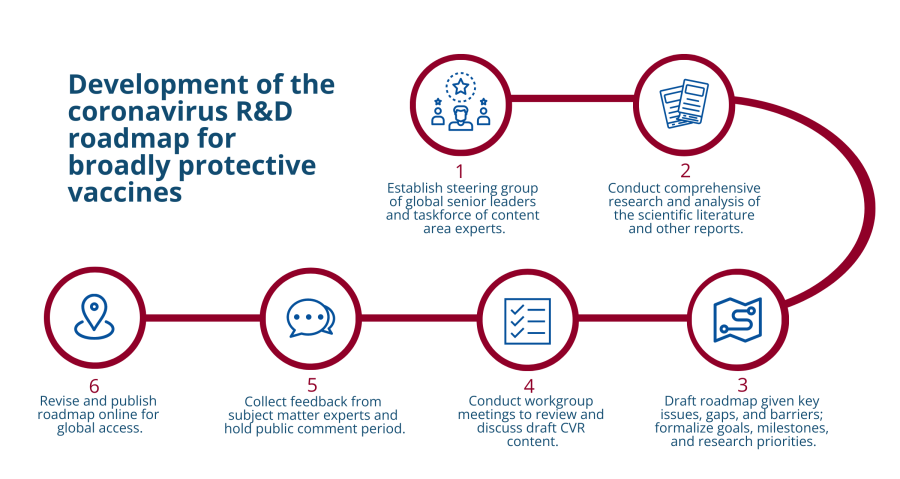
To guide the CVR development process, CIDRAP engaged a small steering group of senior leaders from the Bill & Melinda Gates Foundation; The Rockefeller Foundation; the Wellcome Trust; the National Institute of Allergy and Infectious Diseases (NIAID) of the US National Institutes of Health (NIH); the Coalition for Epidemic Preparedness Innovations (CEPI); several academic institutions (including University of Iowa [USA], University of North Carolina [USA], Icahn School of Medicine at Mount Sinai [USA], and University of the Witwatersrand [South Africa]); and Biologics Consulting, a USA-based consulting firm with expertise in regulatory issues. In addition to the steering group, CIDRAP established a global CVR Taskforce of 39 external SMEs, who offered a wide range of knowledge and experience in vaccine development. This taskforce was charged with providing expert input and commentary on the CVR through detailed online discussions and document review. The CVR was posted on the CIDRAP website for public comment from October 24 to November 18, 2022. Each comment was adjudicated and incorporated into the final roadmap as deemed appropriate by the CIDRAP roadmap development team.
Roadmap Vision
To accelerate the development of durable, broadly protective coronavirus vaccines that: (1) are suitable for use in all regions of the globe, including remote areas and low- and middle-income countries (LMICs), (2) can reduce severe illness and death (and potentially prevent infection) from coronaviruses (both those known to infect humans and pre-emergent viruses), and (3) will mitigate the impact of future coronavirus epidemics or pandemics worldwide.
Roadmap implementation
By identifying R&D priorities for generating broadly protective coronavirus vaccines, the CVR aims to provide a framework to inform the decision-making of funders, researchers, policy makers, and industry leaders. Moving forward, development of an official implementation strategy for the CVR will be needed. It will be essential for key stakeholders to develop a coordinating oversight group or steering committee to accelerate and advance the CVR milestones. Additionally, a system will be needed to monitor and evaluate progress on meeting the CVR goals and milestones over time and to make adjustments as necessary (referred to as a monitoring, evaluation, and adjustment [ME&A] program.
Definition of CVR Terms
Roadmap: a type of strategic plan that identifies key activities over a specified timeframe to achieve the overall objective, which is to advance the development of safe and effective broadly protective coronavirus vaccines.
Barriers: inherent obstacles or technical challenges that may influence the likelihood of success at various stages of coronavirus vaccine development; identifying such barriers helps inform the nature and scope of activities designed to achieve the research and development (R&D) outcomes.
Gaps: key unresolved issues or limitations in knowledge that are critical to the development of new vaccines and that can be addressed through targeted R&D activities.
Strategic Goals: long-range high-level research priorities that the roadmap’s actions are intended to address during the stated timeframe.
Milestones: actions deemed necessary to achieve the roadmap’s strategic goals; the milestones include target dates for completion and reflect SMART (specific, measurable, achievable, realistic/relevant, and time-sensitive) criteria, to the degree feasible.
High-Priority Milestones: milestones that are considered critical for advancing coronavirus vaccine R&D, based on input from the CVR Steering Group and Taskforce.
Additional Research Priorities: further topics and issues that are relevant to the achievement of the strategic goals, but are either not high enough priority to be considered milestones or not sufficiently specific or time-bound to be identified with SMART criteria.
Classification of coronaviruses
Coronaviruses are enveloped positive-sense, single-stranded ribonucleic acid (RNA) viruses that include four genera: alphacoronaviruses, betacoronaviruses, gammacoronaviruses, and deltacoronaviruses. All four genera contain viruses that infect animal species (mainly mammals or birds). Only alphacoronaviruses and betacornaviruses are generally known to infect humans; however, zoonotic spread of porcine deltacoronavirus to humans has recently been described (Lednicky 2021).
- Within the alphacoronavirus genus, two viruses cause illness in humans—human coronavirus 229E and human coronavirus NL63. Both cause mild upper respiratory tract infections consistent with a clinical presentation of the “common cold.” Additionally, a canine alphacoronavirus has been identified as a cause of illness in several people (Zehr 2022).
- Within the betacoronavirus genus, five viruses have been identified that cause human illness. These include SARS-CoV and SARS-CoV-2, MERS-CoV, and two viruses that cause mild upper respiratory tract infections: human coronavirus HKU1 and human coronavirus OC43.
- Gammacoronaviruses to date are not known to infect humans.
- Most deltacoronaviruses also do not infect humans, but human porcine deltacoronavirus strains have recently been identified in plasma samples from several Haitian children with acute undifferentiated febrile illness, suggesting that zoonotic spread of deltacoronaviruses to humans can occur (Lednicky 2021).
Currently, betacoronaviruses are of greatest concern to public health, since this genus includes the three viruses that have caused severe illness and death in humans (SARS-CoV, SARS-CoV-2, and MERS-CoV). Betacoronaviruses include five subgenera: embecoviruses (group 2a), sarbecoviruses (group 2b), merbecoviruses (group 2c), and hibecoviruses and nobecoviruses (group 2d) (Zhu 2020). Human coronavirus HKU1 and human coronavirus OC43 are in the embecovirus subgenus, SARS-CoV and SARS-CoV-2 are in the sarbecovirus subgenus, and MERS-CoV is in the merbecovirus subgenus. The other subgenera (hibecoviruses and nobecoviruses) contain viruses that to date have only been found in animals other than humans and few efforts have been made to characterize these group 2d viruses. The potential for viruses in this group to cause human disease remains unknown and should not be ignored.
Historic occurrence of highly pathogenic betacoronaviruses in humans
SARS-CoV
In November 2002, an outbreak of atypical pneumonia occurred in Guangdong province, China, and additional outbreaks were recognized in that region in early 2003 (Pieris 2003). In February and March 2003, similar outbreaks occurred in Hong Kong, Singapore, and Toronto. SARS-CoV was identified as the causative agent for these outbreaks in March of that year. Over the next few months, the virus spread to 26 countries on five continents, with just over 8,000 cases identified and 774 deaths (Peiris 2003), yielding a CFR of about 10% among identified cases.
Most cases were associated with outbreaks in healthcare settings, although some were associated with “super-spreader” events. In 2004, a second independent spillover of SARS-CoV occurred in China, but only four cases were identified (Wang 2005). Fortunately, the virus typically did not transmit until after the onset of symptoms, which allowed for virus containment through traditional public health and infection control measures. No additional outbreaks of SARS-CoV have been identified since 2004, and the virus has not been found in the animal reservoir since that time, although viruses that are closely related genetically have been found in bats (Ng 2017). Several vaccines targeting SARS-CoV were developed and tested in preclinical models, and a few phase 1 clinical trials were initiated, but no SARS-CoV vaccines have advanced beyond that point (Li 2020).
MERS-CoV
MERS-CoV was first identified in 2012 in a patient from Saudi Arabia who died of atypical pneumonia (Zaki 2012). Since then, cases have continued to occur at a low incidence rate, primarily in the Middle East and particularly in Saudi Arabia. Cases have been identified in 27 countries across the Middle East, North Africa, Europe, North America, and Asia. By November 2022, just over 2,600 cases have been identified globally, with a CFR of about 35% among reported cases (ECDC 2022). Human-to-human transmission (both symptomatic and asymptomatic) occurs; however, transmission is neither efficient nor sustained, so cases have not spread widely. Similar to SARS-CoV, vaccines have been developed and assessed in preclinical models, and several phase 1/2 trials are ongoing (Li 2020).
SARS-CoV-2
SARS-CoV-2, the causative agent of the current pandemic, first emerged in Wuhan, China, in late 2019 and rapidly spread around the globe; the WHO officially declared a COVID-19 pandemic on March 11, 2020 (Cucinotta 2020). As of the end of 2022, more than 650 million cases had been reported worldwide, with more than 6.6 million documented deaths, and these numbers likely underestimate the true burden of disease. The overall global CFR is about 1% among reported cases (WHO 2022a), and may vary somewhat by country and over time (Johns Hopkins University & Medicine 2022). The public health impact of this virus, however, has been much greater, owing to its high transmissibility and the continued emergence of different variants of concern (VOCs) with increased transmissibility and the ability to at least partially evade (at greater or lesser degrees) antibody-induced immune protection from previous infection or vaccination. SARS-CoV-2 vaccines were fast-tracked for development at the start of the pandemic, and vaccines first became available under Emergency Use Authorization in December 2020 (US FDA 2021).
The persistent threat of coronaviruses
Many emerging pathogens in humans originate in wild animal reservoirs. Several factors increase interactions between humans and wild animals, including land-use changes, disruption of natural ecosystems, increased urbanization, travel, climate change, and wildlife trade and consumption (Cunningham 2017, Irving 2021).
As the human population increases, the potential for “spillover” events from zoonotic reservoirs to humans also increases; therefore, we can expect that additional novel viruses, including coronaviruses, will emerge in the future.
Bats are a primary reservoir for several emerging viral pathogens, including Ebola, Nipah, Marburg, and Hendra viruses. Both SARS-CoV and MERS-CoV likely originated in bats, and then later adapted to palm civets (SARS-CoV) and dromedary camels (MERS-CoV) (El Sayed 2021). The source of SARS-CoV-2 has yet to be definitively determined; however, bats, with other animal hosts playing intermediate roles, remain the most likely possibility (Worobey 2022). More than 500 coronaviruses have been identified in various bat species (Chen 2014), and some researchers have estimated that more than 3,000 coronaviruses can be found in bats (Anthony 2017). Horseshoe bats are thought to be a primary reservoir for SARS-related coronaviruses in Russia and China (Hu 2017, Alkhovsky 2022). Additionally, bats are considered to be the major evolutionary reservoir and ecological driver of coronavirus diversity globally (Anthony 2017). Given that coronaviruses can evolve rapidly, and that many are receptor generalist viruses, we can expect that pathogenic coronaviruses will emerge from the bat reservoir or some intermediate host in the future (El Sayed 2021).
Sarbecoviruses often undergo recombination, which can have evolutionary advantages. For example, researchers have postulated that the emergence of SARS-CoV resulted from a recombination event within an animal host that allowed the virus to bind to the human angiotensin-converting enzyme 2 (hACE2) receptor site on epithelial cells, which is the primary target for viral entry (Wells 2021). The ability of sarbecoviruses to infect multiple host species in addition to bats creates opportunities for coinfection, mutation, and recombination, which can result in the emergence of novel sarbecoviruses with pandemic potential (Wells 2021, Ren 2008). However, since SARS-like strains that are genetically very similarity to SARS-CoV and SARS-CoV-2 are circulating in nature, the persistent threat already exists and does not necessarily depend on a future random recombination event.
Recent research demonstrates that SARS-CoV-2 can infect multiple animal species in natural settings, including dogs, domestic cats, large wild cats (tigers, lions, etc.), gorillas, ferrets, mink, and white-tailed deer (Goraichuk 2021, Meisner 2022, Pickering 2022, Sharun 2021, Tan 2022, Telenti 2022). Based on the presence of angiotensin-converting enzyme-2 (ACE2) receptors in host species, other animals may also be at risk of infection. Given the potential of the virus to jump species, the possibility exists for SARS-CoV-2 to undergo recombination with other coronaviruses, thereby generating a novel virus with renewed pandemic potential. Moreover, humans can spread the virus to other species, such as domestic pets, mink, and deer (reverse zoonotic transmission or zooanthroponosis).
Coronaviruses have the potential to be highly pathogenic in humans, as illustrated by the approximately 35% CFR for MERS-CoV and the 10% CFR for SARS-CoV. Fortunately, MERS-CoV and SARS-CoV spread less efficiently between humans than SARS-CoV-2; however, we cannot rule out the possibility that a highly pathogenic and highly transmissible coronavirus could emerge from a bat or intermediate host in the future. Given the ongoing threat posed by coronaviruses, broadly protective vaccines are needed to protect against the emergence of additional SARS-CoV-2 variants and future novel coronaviruses with pandemic potential.
Strategies for development and use of broadly protective coronavirus vaccines
Protection against infection versus protection against severe disease
An important consideration for R&D of broadly protective coronavirus vaccines is defining what is meant by “protection.” Ideally, future coronavirus vaccines would protect against infection and, in doing so, would not only prevent disease, but would also block transmission. This approach would decrease the level of circulating viruses in the population. Existing vaccines for SARS-CoV-2 often do not protect against infection (depending in part on the vaccine, how long since the last dose, and the variant exposure), but rather primarily protect against severe disease and death (Wahl 2022). This allows SARS-CoV-2 viruses to continue to circulate, which in turn can lead to viral mutagenesis and recombination, and the potential for new VOCs to emerge. Creating transmission-blocking vaccines is challenging, however, and may require strong mucosal immune responses in both the upper and lower respiratory tracts. As preferred product characteristics for next-generation coronavirus vaccines are defined, it is likely that transmission blocking will be considered aspirational or optimal, while preventing severe disease and death will continue to be the more realistic goal.
Breadth of protection
Researchers have several options to consider when developing broadly protective coronavirus vaccines, including the following:
- “Variant-proof” SARS-CoV-2 vaccines: These vaccines would protect against all SARS-CoV-2 variants—those that have emerged and those that could emerge in the future.
- Vaccines that protect against a wide range of sarbecoviruses: These vaccines would include protection against SARS-CoV and SARS-CoV-2 variants and potentially against other novel sarbecoviruses.
- Vaccines that protect against a wide range of betacoronaviruses: These vaccines would protect against betacoronaviruses, including SARS-CoV, SARS-CoV-2, MERS-CoV, those that cause mild illness, and “pre-emergent” betacoronaviruses in zoonotic reservoirs that could spill over into humans.
- Vaccines that protect against a wide range of all coronaviruses (also referred to as “universal” coronavirus vaccines): Such vaccines would protect against representative viruses from all of the coronavirus genera, including the milder “common cold” species and novel pre-emergent coronaviruses with pandemic potential.
Although betacoronaviruses are currently of greatest concern, the potential for alphacoronaviruses or other coronaviruses to cause serious human disease should never be minimized. A stepwise approach for vaccine development, therefore, may be the most practical strategy. This approach would start with the highest-priority viruses and then gradually expanding coverage over time, as new scientific information and technological solutions become available. For example, vaccines against SARS-CoV-2 variants would be the highest priority, followed by vaccines against all sarbecoviruses, then merbecoviruses, then all betacoronaviruses, and finally, vaccines that protect against a wide range of coronaviruses from all four genera.
When designing broadly protective coronavirus vaccines applicable to one or more of the categories outlined above, different approaches are possible. For next-generation SARS-CoV-2 vaccines, a primary strategy is to identify immunogens that generate broadly neutralizing antibodies against conserved regions of SARS-CoV-2 variants. Such vaccines can potentially capitalize on the fact that SARS-CoV-2 viruses bind primarily to the hACE2 receptor on human epithelial cells. Host-cell binding is mediated through the receptor binding domain (RBD) on the virus spike (S) glycoprotein, which appears to be relatively immunodominant, and neutralizing antibodies to this area appear to inhibit receptor attachment in the host—although there is a large mutational space in the RBD that can escape antibodies but still retain ACE2 binding activity.
The search for broadly protective sarbecovirus vaccines is complicated by the fact that not all sarbecoviruses use hACE2 as the host receptor (Wells 2021). However, there may be other immunogenic epitopes on the S protein (e.g., within the RBD, the N-terminal domain [NTD], or subdomains of the S1 subunit or the S2 subunit) that are shared across sarbecoviruses; therefore, additional efforts to identify such epitopes are warranted (Yuan 2020). Several recent studies, for example, found that a SARS-CoV-2 RBD and spike nanoparticle with an adjuvant elicited cross-neutralizing antibody responses against SARS-CoV, several SARS-CoV-2 variants, and several bat coronaviruses (Joyce 2022, Saunders 2021). Another recent study in hamsters and mice found that immunization with an S2-based nanoparticle vaccine elicited broadly cross-reactive antibodies to SARS-CoV-2 variants, SARS-CoV, and the four endemic human coronaviruses (Halfmann 2022). Additionally, Fc receptor (FcR)-mediated cross-protective immune responses may be critical in universal pan-sarbecovirus vaccine designs (Adams 2022, Mackin 2022). An alternative approach for developing broadly protective sarbecovirus vaccines is to generate vaccines that contain multiple representative immunogens from different clades within the sarbecovirus subgenus, such as through development of chimeric spike vaccines or mosaic/multiplexed nanoparticle vaccines (Cohen AA 2021, Cohen J 2022, Martinez 2021, Walls 2021, Wuertz 2021). This strategy is also under investigation for generating broadly protective influenza vaccines (Arevalo 2022, Pecetta 2022a). Prime-boost strategies using different immunogens may offer a third option toward creating broadly protective sarbecovirus vaccines (Tan 2021). Finally, use of T-cell epitopes and non-structural proteins as immunogens may be another option.
Similar approaches can be used for developing broadly protective betacoronavirus vaccines. One recent study, for example, identified a monoclonal antibody that cross-reacts with the S glycoproteins from eight betacoronaviruses, including all five betacoronaviruses known to be pathogenic in humans (Sauer 2021). Studies such as this suggest that it may be possible to identify epitopes that are broadly protective and immunogenic across different genera of coronaviruses. Alternatively, multivalent approaches that combine immunogens from different virus groups may enable the development of vaccines that are broadly protective across genera.
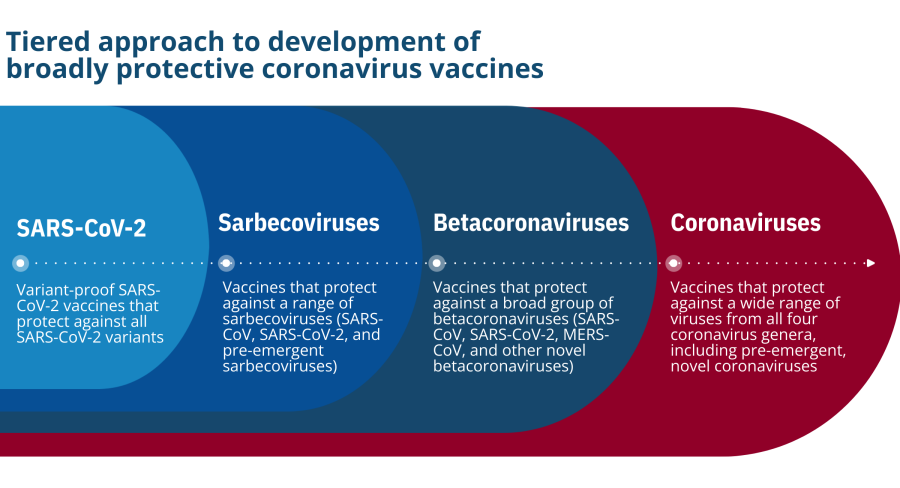
Use of broadly protective coronavirus vaccines
Several strategies can be considered for using broadly protective coronavirus vaccines.
- The most expansive approach is to use broadly protective coronavirus vaccines as part of routine childhood or adult vaccination programs (prophylactic use). This strategy would be an option if vaccines that are more durable can be developed (i.e., durability of a year or more), and may be important if new SARS-CoV-2 variants that cause significant morbidity and mortality continue to circulate over time in the global population. This approach also allows for sequential boosting by naturally circulating contemporary SARS-CoV-2 strains and other coronaviruses.
- Another approach is to use these vaccines to enhance pandemic preparedness by having vaccines available that will protect against novel coronaviruses with pandemic potential if such viruses emerge from an animal reservoir (reactive use). Such vaccines could be stockpiled in sufficient quantities for early use at the onset of an outbreak to rapidly interrupt transmission and prevent escalation into a pandemic, with production to be scaled up quickly as needed. This approach limits the lag time necessary to generate a new vaccine.
- A third option involves a hybrid of the previous two, where routine prophylactic vaccine use could be recommended for certain groups at high-risk of exposure to novel or existing coronaviruses or for individuals at high risk of severe disease, whereas reactive use could be reserved for the general population if a novel virus emerges and spreads. For example, healthcare personnel and animal workers in regions where MERS-CoV is enzootic could be considered for routine vaccination with a broadly protective vaccine that protects against that virus.
As these vaccines are developed, certain key attributes should be considered during the R&D process. Examples include the following: (1) affordable and suitable for use in all regions of the world, including LMICs; (2) ability to prevent severe disease, protect against all sarbecoviruses and merbecoviruses, and elicit rapid and robust immune responses; (3) immunogenic in persons with preexisting immunity; (4) a safety profile that is acceptable to the public; (5) suitability in all age-groups, immunocompromised people, pregnant women, and other special populations (Morens 2022); (6) durability (for at least 1 year); and (7) effective with one dose (or only a few doses). Ability to block transmission is another desirable attribute, but additional scientific discovery is needed to generate transmission-blocking vaccines (Morens 2022).
This section summarizes the virology issues related to development of broadly protective coronavirus vaccines. An improved understanding of the global distribution of coronaviruses in humans and animal reservoirs worldwide, using standardized tools and methods, is needed to guide a well-informed R&D strategy. Coordinated and sustained efforts that collect, characterize, and share viral information will accelerate progress toward vaccine development.
Issue: Coronaviruses are globally distributed and the coronavirus universe has not been well characterized.
Barriers
- Coronaviruses have the capacity to readily transmit within and between a wide, yet not fully defined, range of hosts (Millet 2021, Morens 2022, Singh 2021). Owing to their expansive presence in various geographic settings and diverse host species, efforts to better characterize this virus family through sampling and sequencing are inherently complicated (Ghai 2021, Morens 2022, Terrier 2021).
- Bats (and possibly rodents to a lesser degree) are considered the primary zoonotic reservoir for coronaviruses, with other species likely serving as intermediate hosts (Frutos 2021, Sánchez 2022). Bats are found on six of the seven continents and are the second most diverse order of mammals, with more than 1,400 species identified. Moreover, more than 500 coronavirus species have been found in bats (Chen 2014), and researchers suggest that the actual number may be more than 3,000 (Anthony 2017). This remarkable diversity and wide geographic range create major challenges for efforts to globally characterize coronaviruses within the bat reservoir (Lattine 2020, Ruiz-Aravena 2022).
- In addition to bats and rodents, a wide variety of other mammals and birds likely harbor novel undiscovered coronaviruses, which further complicates efforts to understand the coronavirus universe.
- SARS-CoV-2 has been transmitted from humans to a number of animal species, such as mink, white-tailed deer, domesticated pets (i.e., cats and dogs) and large cats (Goraichuk 2021, Meisner 2022, Pickering 2022, Tan 2022, Telenti 2022), which adds another layer of complexity to understanding the coronavirus ecosystem.
- Evidence for circulation of merbecoviruses has been found in all regions that have dromedary camel holdings, but spillovers to humans have mostly been observed in countries on the Arabian Peninsula, although spillover has also likely occurred in Africa (Zhou 2021). One possible explanation for the varying potential for spillover is the presence of genetically and phenotypically diverse MERS coronaviruses in different regions (Zhou 2021, Zhou 2023), but more work is needed to understand the diversity of MERS coronaviruses and their zoonotic potential.
- Efforts to further define the coronavirus universe are important for global health and security; however, research involving potential pandemic pathogens elicits questions and concerns about biosafety and biosecurity. These risks must be considered and appropriately mitigated to ensure safe research environments (US DHHS 2017).
Gaps
- While recent efforts have been undertaken to expand sampling of wild and captive animals for coronaviruses, further work is needed to improve understanding of the geographic distribution, viral diversity, host range, and prevalence of this family of viruses, and to link such information to human surveillance data, and ultimately to vaccine R&D (Baric 2022, Keusch 2022, MacLean 2021, Morens 2022, Terrier 2021).
- Although bats may be the primary reservoir, other animal hosts may play an important intermediate role between bats and humans (Ghai 2021, Terrier 2021); therefore, an improved understanding is needed of intermediate animal reservoirs to better define the risk of spillover events to humans (Morens 2022, Ruiz-Aravena 2022, Terrier 2021).
- Further identification and characterization of diverse coronaviruses is needed to guide a coordinated, well-informed process of virus strain selection for research aimed at broadly protective coronavirus vaccine development (Baric 2022).
- To achieve this, a key consideration is to determine the degree of phylogenetic and antigenic diversity of strains necessary to ensure adequate breadth of coverage for vaccine R&D. Therefore, obtaining viruses from the different genera will be necessary to obtain representative sampling of coronaviruses that have potential for spillover into human populations.
- Betacoronaviruses are considered to be at high risk for spillover; therefore, research campaigns are particularly needed to better characterize these viruses. Group 2d betacoronaviruses (hibecoviruses and nobecoviruses) are generally not available for study, and virus stocks of group 2c betacoronaviruses (merbecoviruses) are limited. This situation must be remedied to develop effective broadly protective betacoronavirus vaccines.
- Availability of a wide range of coronaviruses for study can promote the discovery and characterization of conserved B- and T-cell epitopes that exist within different coronavirus species, which may be a critical issue for R&D of broadly protective vaccines (Baric 2022, Morens 2022, Starr 2021). (See Immunology and Immune Correlates of Protection.)
- Serologic studies are important to improve understanding of the frequency and scale at which exposure to coronaviruses occurs in various species and geographic settings. Serosurveys of wild and captive animals could uncover potential reservoirs, which would inform subsequent risk assessments. Serosurveys in human populations, particularly those living or working in close contact with known and potential animal reservoirs, would enhance the understanding of exposure frequency and associated risk factors (Morens 2022, Ruiz-Aravena 2022, Sánchez 2022).
- A limitation in culturing and studying bat-derived coronaviruses in the laboratory is an overall lack of accessible reagents (such as cell-culture reagents that can facilitate virus isolation) and diverse cell lines that are readily susceptible to an array of bat-derived coronaviruses (Letko 2020a, Ruiz-Aravena 2022).
- An additional limitation is the lack of available biosafety level (BSL)-3 facilities for use by academic investigators.
- Coordinated funding programs and resources to conduct the necessary research campaigns are needed to enhance scientific discovery in this area. Given the diversity of coronaviruses in different regions, such programs should include investment in building research capacity in LMICs.
Issue: Coronaviruses frequently undergo mutation and recombination, which complicates understanding and tracking host range and viral spread.
Barriers
- The wide geographic distribution of coronaviruses, the broad range of hosts, the specific ecology and behavior of bat populations, and the large genome size offer ample opportunity for coronaviruses to undergo mutation and recombination (Morens 2022, Terrier 2021, Zhu 2020, Forni 2017, Kistler 2021, Millet 2021). This overall propensity to tolerate change also applies to the spike protein (particularly the S1 subunit), ultimately enabling the possibility of distinct modifications to occur within or near antigenic sites without sacrificing viral fitness (Cotten 2021, Telenti 2022).
- The co-circulation of distinct coronaviruses among host species in the same geographic area, and the sharing of habitats by different host species such as bats, increases the likelihood of co-infections and subsequent recombination events (Latinne 2020, Ruiz-Aravena 2022, Wells 2021). Evidence suggests that certain bat populations—which can live in large colonies and share densely populated roosts with other species—frequently experience co-infections involving one or more coronaviruses (Ruiz-Aravena 2022). Co-infections can facilitate rapid viral adaptation to new hosts and ecologic environments (Forni 2017, Telenti 2022, Woo 2009).
- Selective pressures on at least several human coronaviruses—including OC43, 229E, and SARS-CoV-2—are dynamic and capable of altering antigenic sites (Cameroni 2022, Eguia 2021, Kistler 2021).
- Current capacity for phenotypic characterization of coronaviruses is limited by the lack of available tools, the high-level technical expertise required to perform such work, its time-consuming nature, and the associated costs (Letko 2020a, Letko 2020b). In turn, the overall lack of functional characterization restricts the interpretation of genomic sequencing data and delays understanding of the viral factors associated with traits such as zoonotic potential and virulence (Letko 2020a, Telenti 2022).
- Global surveillance and sharing of information, samples, and reagents is hampered by legislative procedures that differ in different parts of the world. The introduction of the Nagoya protocol with different interpretations in different regions has extended this to ecological sampling (CBD 2011, Ribeiro 2018).
Gaps
- A robust panel of small and large animal models of human disease is needed, including models that recapitulate severe human acute and chronic disease.
- Implementation of a collaborative, long-term effort to conduct genomic sequencing of coronaviruses from various animal species across multiple, diverse regions of the globe is needed to inform coronavirus surveillance and risk assessment to identify coronaviruses with pandemic potential. Generating viral sequencing data that are open, accessible, standardized (including metadata), and thorough would permit high-throughput analyses that could ultimately help bridge phylogenetic gaps present in the coronavirus virome and illustrate the diversity that exists across different populations and geographic settings (Baric 2022, Chen 2022, Morens 2022).
- Investment in resources and global initiatives that expedite the functional characterization of coronaviruses is important for deciphering the relationship between genotype and phenotype and identifying genetic markers that can alter—and potentially enhance—characteristics such as transmissibility, immune evasion, and virulence (Forni 2017, Letko 2020a, Obermeyer 2022, Terrier 2021, Oude Munnink 2021).
Issue: SARS-CoV-2 variants of interest, concern, and high consequence will likely continue to emerge, and expanded efforts are needed to track viral phylogenetic evolution in real time and over time.
Barriers
- The continued circulation and adaptability of SARS-CoV-2 over time has manifested in the emergence of multiple VOCs and descendent subvariants. The VOCs that have emerged to date have done so independently, with each leveraging the characteristics conferred by its distinct constellation of mutations to outcompete previously circulating variants (Obermeyer 2022, Telenti 2022).
- The SARS-CoV-2 spike protein has a high proclivity for significant changes in antigenic sites, with multiple VOCs and descendent subvariants having noticeable impact on the effectiveness of available vaccines and treatments, particularly in preventing or treating less severe disease (Hachmann 2022, Mannar 2022).
- Growing immunity in the population, achieved through vaccination or previous infection, has potentially placed selective pressure on antigenic evolution (Harvey 2021, Markov 2022, Yewdell 2021a).
- Persistent infections, particularly in immunocompromised hosts, may contribute to the emergence of new variants through intra-host recombination or viral evolution (Simons 2022).
- Existing disparities among countries and global regions in systems infrastructure, expertise, human and financial resources, and overall sequencing and surveillance capacity constrain the implementation of coordinated and uniform efforts to improve SARS-CoV-2 global genomic surveillance (Chen 2022, Houtman 2022). Even if infrastructure, funding, and expertise are available, wide variations in technology used for such ventures can slow data turnaround times, and the associated costs can limit capacity.
- Research involving SARS-CoV-2 and other emerging coronaviruses must be conducted in BSL-3 labs, which creates challenges. In addition, biosafety levels fluctuate among countries, indicating the need to harmonize biosafety regulations.
- Genomic surveillance data for SARS-CoV-2 are available through the Global Initiative on Sharing All Influenza Data (GISAID), the International Nucleotide Sequence Database Collaboration (INSDC), and other platforms, but the data are not necessarily accurate, standardized, or thorough. INSDC also contains data for other coronaviruses and has metadata standards, but the information often is not complete.
- Countries may not be willing to share coronavirus genomic or prevalence data quickly with the global scientific community because of concerns about public image, the potential for border closings, and economic implications (Mendelson 2021, Ribeiro 2018).
- Widespread and persistent circulation of SARS-CoV-2 in both human and animal populations elicits the theoretical possibility of recombination with other coronaviruses (Telenti 2022), which could propagate viruses with unanticipated characteristics. Furthermore, sustained circulation of SARS-CoV-2 among a range of wild and domestic animals presents the risk of long-term reservoirs that could result in divergent or recombinant strains and spillback into humans (Peacock 2021, Pickering 2022, Rabalski 2022, Sila 2022).
- The continued circulation of MERS-CoV in camels in two diverse geographic regions—the Arabian Peninsula and Africa—also offers opportunity for further evolution and diversification, with potential zoonotic spillover and adaptation risk.
- The lack of a standardized nomenclature for variants, coupled with the fact that sequences are being made available on several databases and platforms without consistency across systems and without release of the raw information, obfuscates the interpretation and representativeness of available sequencing data for SARS-CoV-2 (Chen 2022, Lancet 2021).
Gaps
- While progress occurred during the COVID-19 pandemic in expanding global capacity to conduct genomic sequencing for SARS-CoV-2 (particularly in LMICs and other low-resource settings), more needs to be done in real time to generate meaningful genomic surveillance, obtaining a more comprehensive and representative understanding of SARS-CoV-2 distribution and evolution, and understanding the impact of antigenic changes. Data generated from such initiatives are important for evaluating the effectiveness of available SARS-CoV-2 vaccines and ensuring that future vaccine candidates will protect against antigenically drifted variants.
- In areas where this capacity already exists (including expansion since the emergence of COVID-19), systems should be maintained and a greater understanding is needed of the specific barriers and bottlenecks that limit sequencing and data sharing (Babady 2022, Keusch 2022). Furthermore, strategies are needed to build laboratory capacity that is integrated with existing programs to improve systems while also preserving limited resources. Recent information indicates that SARS-CoV-2 surveillance is declining around the globe, which will leave the world unprepared to face new SARS-CoV-2 variants or to prepare for the next pandemic (Economist 2023).
- Establishing the upload of raw, standardized genomic sequencing data and metadata to public databases as a norm, whenever possible, would enhance the ability to accurately interpret sequencing data, critically evaluate data sets, and provide opportunities for quality assurance.
- The effect, if any, of the Nagoya Protocol (CBD 2011), on virus sharing and the advancement of novel coronavirus vaccines should be assessed over time, including the impact of national Access and Benefit Sharing legislation (Babady 2022, Mueni Katee 2021, WHO 2017).
- Efforts to expand the use of computational and machine learning tools for analyzing genome sequence data sets can improve capabilities for predicting SARS-CoV-2 virus evolution, which could assist in vaccine R&D aimed at broadly protective coronavirus vaccines (Telenti 2022).
- The open sharing of genomic data and linked phenotypic data should be widely encouraged (Harrison 2021).
Issue: Coronaviruses can bind to different cell receptors, and the breadth and specificity of host-cell receptors has not been fully elucidated.
Barriers
- Coronavirus spike proteins are capable of binding to a diverse array of cell receptors in both animals and humans, which helps facilitate their broad host ranges (Forni 2017, Kistler 2021, Millet 2021, Tan 2022, Zmasek 2022). For example, SARS-CoV and SARS-CoV-2 utilize the ACE2 receptor and MERS-CoV uses the dipeptidyl peptidase 4 (DPP4) receptor. For a number of coronaviruses, the host-cell receptor has yet to be characterized.
- Receptor binding appears to be an evolvable trait, with analyses suggesting that SARS-CoV-2 may have obtained its ability to use hACE2 through recombination (Tan 2022, Wells 2021, Xiong 2022) or perhaps through evolution from common ancestral strains. Notably, evidence exists of non–ACE2-using coronaviruses circulating in the same geographic areas with ACE2-using coronaviruses, which poses the risk of shifting receptor usage and altering host ranges. Recently, MERS-CoV–related viruses have been identified that use the hACE2 receptor, rather than DPP4, which underscores the “promiscuity” of receptor use and highlights the ongoing potential zoonotic threat of these viruses (Xiong 2022). In addition, many sarbecovirus RBDs can acquire the ability to bind to select ACE2 receptors from a single amino-acid change (Starr 2022). Ongoing evolution of SARS-CoV-2 has demonstrated this malleability, with broadened host-cell receptor tropism periodically linked to emerging VOCs (Shuai 2021).
- In addition to cell-receptor binding, an undefined array of additional host-cell factors, such as proteases, often play a significant role in viral entry (MacLean 2021, Millet 2021).
Gaps
- Further research is needed to:
- Identify the main host-cell receptors to which different coronaviruses bind (Ghai 2021). In particular, defining the range of ACE2-using coronaviruses could improve capacity to assess zoonotic risk (Wells 2021). For example, coronaviruses identified in bats and pangolins have RBDs closely resembling that of SARS-CoV-2, which can readily bind to hACE2 (Holmes 2021, Seifert 2022, Telenti 2022, Temmam 2022). Further understanding of the full range of host receptor binding is important for developing broadly protective vaccines.
- Determine the presence or absence of host-cell receptors and additional factors, such as proteases, important for viral entry and map their distribution across different species and in different tissue types to determine tissue tropism (Cervantes 2022, Hu 2021, Millet 2021, Ruiz-Aravena 2022).
Strategic Goals and Aligned Milestones for Topic 1: Virology Applicable to Vaccine R&D
Strategic Goal 1.1: Enhance and sustain the capacity to identify, characterize, and share SARS-CoV-2 variants of interest, concern, and high consequence among researchers globally. |
Milestone 1.1.a: By 2023, initiate the risk assessment and decision-making processes necessary to determine if some SARS-CoV-2 lineages or clones can be safely reclassified from BSL-3 to BSL-2 and under what conditions. |
Milestone 1.1.b: By 2023, develop a strategy to ensure that the global capacity developed during the COVID-19 pandemic to conduct genomic sequencing of SARS-CoV-2 viruses sampled from humans can be maintained over time, particularly in low-resource settings. |
Milestone 1.1.c: By 2023, standardize SARS-CoV-2 genomic sequencing data and metadata (including nomenclature) aimed at enhancing accurate interpretation and use. |
Milestone 1.1.d (High Priority): By 2024, generate a financially sustainable, collaborative international program to quickly identify, characterize, and share information on SARS-CoV-2 viruses, including antigenic information, in real time with the potential to build on current systems such as the WHO’s Global Influenza Surveillance and Response System (GISRS) (WHO 2022b, Harvey 2021, Subbarao 2021). |
Strategic Goal 1.2: Improve characterization of the coronavirus universe to determine the diversity of strains necessary to ensure adequate breadth of coverage for vaccine R&D. |
Milestone 1.2.a: By 2024, establish international best practices and standard operating procedures for research in the field and in the laboratory involving coronaviruses of unknown pathogenicity to ensure that biosafety and biosecurity risks are minimized. |
Milestone 1.2.b: By 2024, initiate research campaigns aimed at: (1) identifying additional bat-derived coronaviruses (particularly group 2d betacoronaviruses) and (2) generating critical reagents needed to study such viruses. |
Milestone 1.2.c (High Priority): By 2024, devise a consensus approach to prioritize and select coronaviruses that would constitute an optimally diverse panel to be used in vaccine R&D for assessing breadth of protection (Baric 2022). Selection criteria should initially focus on alphacoronaviruses and betacoronaviruses that: (1) use the hACE2 receptor, (2) grow in primary human cells, (3) are genetically diverse, (4) have been antigenically characterized, and (5) have strains available for study. |
Milestone 1.2.d: By 2024, develop a coordinated international framework to enhance sampling of wild and captive animal populations—particularly bats—in geographically diverse regions for improving understanding of the distribution, viral diversity, host range, and prevalence of coronaviruses globally (Baric 2022, Morens 2022, Terrier 2021). |
Milestone 1.2.e: By 2024, ensure availability of reagents, such as reference monoclonal antibodies for antigen characterization, necessary for evaluating priority coronaviruses, which include sarbecoviruses (group 2b) and merbecoviruses (group 2c) (Letko 2020a, Ruiz-Aravena 2022). |
Milestone 1.2.f (High Priority): By 2024, generate at least one initial panel of virus stocks featuring different coronaviruses and diverse cell lines that are readily susceptible to a wide range of coronaviruses, and make the panel accessible to researchers working on coronavirus vaccine R&D (Letko 2020a, Ruiz-Aravena 2022). |
Milestone 1.2.g: By 2025, develop the serologic platforms needed for conducting serosurveillance studies in high-risk populations (based on a diverse panel of coronaviruses that may pose a risk to human health) to identify signals suggesting the potential for spillover from animals to humans. |
Milestone 1.2.h: By 2025, establish a global framework for serosurvey methodologies—including populations to study—to synchronize study designs. |
Strategic Goal 1.3: Improve understanding of the phylogenetic evolution over time of animal-derived coronaviruses. |
Milestone 1.3.a: By 2024, initiate and implement a collaborative, coordinated, and sustainable effort to conduct genomic sequencing of coronaviruses from relevant animal species sampled across multiple regions of the globe and ensure that the generated viral sequencing data are openly accessible with standardized metadata (Baric 2022, Chen 2022, Morens 2022). |
Strategic Goal 1.4: Improve understanding of the breadth of host-cell receptors for coronaviruses. |
Milestone 1.4.a: By 2026, identify the host-cell receptors to which a sample of different coronaviruses bind, with an initial focus on priority viruses, such as betacoronaviruses, to determine the species distribution for different receptors (Ghai 2021). |
Milestone 1.4.b: By 2027, once host-cell receptors are identified for different coronaviruses, determine which are present in humans (Hu 2021, Millet 2021, Ruiz-Aravena 2022). For those that are in humans, assess the distribution across various tissue types in both humans and commonly used animal models to determine tissue tropism. |
Additional Research Priorities
- Continue to obtain additional SARS-CoV-2 isolates in real time and over time and ensure that these isolates are made equally accessible to suitable researchers, which could broaden phenotypic characterization.
- Perform additional analyses of endemic seasonal human coronaviruses to further understand the pathways and mechanisms of coronavirus evolution.
- Conduct ongoing high-throughput analyses of genomic sequence data for diverse coronaviruses to bridge phylogenetic gaps present in the coronavirus universe and improve understanding of the antigenic diversity of these viruses.
- Update the supply of necessary reagents routinely as additional viruses are identified.
- Expand the use of computational and machine-learning tools for genomic sequence data sets to improve capabilities for predicting SARS-CoV-2 virus evolution.
- Ensure that raw genomic sequencing data on SARS-CoV-2 sequences are readily and widely accessible whenever possible.
- Expand the functional characterization of coronaviruses to improve understanding of the relationship between genotype and phenotype of coronaviruses (Forni 2017, Letko 2020a, Obermeyer 2022, Terrier 2021).
- Continue to build global infrastructure and capacity for conducting virologic surveillance, particularly in LMICs.
- Continue to assess the effect, if any, of the Nagoya Protocol on virus sharing and on the advancement of novel coronavirus vaccines.
This section summarizes the advances needed in the science of human immunology to develop broadly protective coronavirus vaccines. Key areas of research include identifying factors that expand the breadth and durability of immune protection; characterizing the mechanisms involved in systemic and mucosal immunity that protect against coronavirus infection, disease, and transmission; and identifying immune correlates of protection. Standardization of assays, specimen collection and research methods, improved information sharing, and creation of virtual biorepositories will facilitate collaboration and accelerate scientific discovery.
Issue: An improved understanding is needed regarding the mechanisms of mucosal and systemic immunity relevant to SARS-CoV-2 infection and the development of broadly protective coronavirus vaccines.
Barriers
- Innate and adaptive immune responses to SARS-CoV-2 and other coronaviruses involve complex, interrelated physiologic mechanisms and biomarkers that are inadequately understood. Fundamental questions remain concerning the nature of protective and cross-protective immunity to coronavirus infection and to immunity elicited by vaccination (Aguilar-Bretones 2023, Diamond 2022, Siggins 2021).
- Various host and environmental factors, such as age, sex, comorbidities, and geographic location, influence protective immune responses to viral antigens, which can complicate research on broadly protective coronavirus vaccines (Tomalka 2022).
- Mucosal immunity is likely to be important for protection against coronavirus infection and transmission, since coronaviruses are respiratory pathogens that do not have obligate viremic spread (i.e., dissemination from the initial infection site via lymph and blood as a required step in pathogenesis or transmission) (Yewdell 2021b, Morens 2023). This creates a number of important challenges, since the role of mucosal immune protection is not well elucidated, nor are the strategies to stimulate and measure mucosal immunity (Iwasaki 2016, Lavelle 2022, Mao 2022, Morens 2023).
- Obtaining appropriate and adequate clinical samples for studying mucosal and systemic immunity related to coronavirus virus infection can be challenging (Logue 2022).
Gaps
- A greater understanding is needed of innate and adaptive immunity, which is critical for developing vaccines to control respiratory infections such as COVID-19 (Sette 2021), particularly with regard to preventing severe disease, but also reducing symptomatic illness and transmission. Specifically, information is needed to clarify the following:
- How innate immunity influences adaptive (B-cell and T-cell) immune responses to SARS-CoV-2 infection, such as determining the signaling pathways underlying establishment of long-lived plasma cells, memory B cells, and memory T cells (Tomalka 2022, Sette 2021).
- The potential for improving breadth of protection against coronaviruses by stimulating innate “trained” immunity (Mettelman 2022, Verbeke 2022, Ziogas 2022).
- The role of the three main components (B cells, CD4 T cells, and CD8 T cells) of adaptive immunity to SARS-CoV-2 virus infection and vaccination (and to other coronaviruses), with a focus on their specific functions and kinetics (Moss 2022, Sette 2021, Sette 2022, Wherry 2022). This includes a specific focus on the role of key subpopulations, such as T follicular helper cells, regulatory T cells, memory T cells, and memory B cells (Kent 2022, Moss 2022, Tarke 2022, Yu D 2022, Zheng 2021).
- The role and mechanisms of adjuvants in mediating interactions between innate and adaptive immune responses (Carmen 2021, Lee 2022) (e.g., driving breadth of response via CD4 T-cell activation) (Joyce 2022).
- The relative roles of mucosal versus systemic immunity in protecting against coronavirus infection and limiting the potential for virus transmission (Mettelman 2022, Mostaghimi 2022, Poland 2021).
- The role of B cells for viral clearance, limiting symptomatic or severe disease, generating robust immune memory, and generating a protective immune response to different viral variants (Qi 2022).
- Protective immunity mediated by antibodies, including thresholds for protective levels of neutralizing antibody responses, potential roles of non-neutralizing antibodies, and mechanisms of coordination among B- and T-cell components (Khoury 2022).
- The role of T cells for: viral clearance, preventing infection in the absence of seroconversion, limiting the extent of disease following infection, generating robust immune memory, and responding to different viral variants (Grifoni 2022, Wherry 2022).
- The role of antibodies, B cells, and T cells in reducing transmission risk (van Kampen 2021).
- Defining the features of an optimal coordinated cellular immune response to primary SARS-CoV-2 infection and determining the optimal vaccine-elicited cellular immune responses needed to reduce severe disease and prevent transmission, which could in turn prevent the emergence of new viral variants (Moss 2022).
- The immune responses to different vaccine constructs, including in the context of hybrid immunity, and strategies for administering them, including different routes such as intranasal, oral, inhaled, transdermal, and intramuscular administration. Of particular interest is tissue resident memory (TRM) cells in B- and T-cell populations in the upper and lower respiratory tracts (Mettelman 2022, Nelson 2021).
- Immune mechanisms underlying potential vaccine-associated enhancement of disease (VAED), such as eosinophilic immunopathology, in the context of SARS-CoV-2 infection or vaccination, given historical evidence for VAED in some rare circumstances with other viruses (Bigay 2022, Bolles 2011, DiPiazza 2021, Gartlan 2022, Munoz 2021). This may be particularly important for vaccines that elicit antibody responses beyond what has been tested to date for SARS-CoV-2 vaccines.
- The processes by which immune dysregulation may contribute to severe COVID-19 disease following infection and the implications for development of next-generation vaccines, particularly with regard to determining the role that CD8 T-cell responses or the innate immune response may play in stimulating pro-inflammatory reactions or enhanced immunopathology (Ahmed-Hassan 2020, Zheng 2022).
- Immunologic assays, including high-throughput neutralization assays, T-cell assays, and memory B-cell assays—that at a minimum are qualified and ideally are validated—are needed to study immune responses generated by broadly protective coronavirus vaccines (Baric 2022, Goldblatt 2022a, Vardhana 2022). (See Vaccinology.)
- Biomarkers for innate immunity are needed to evaluate and predict mechanisms of the adaptive immune response to coronavirus infection (Espinoza 2022).
- Systemic surrogates of mucosal immunity are needed to accelerate the development of mucosal coronavirus vaccines (Matuchansky 2021).
- Standardized assays for rapid assessment of key traits of emerging variants for use in laboratories across the globe.
Issue: The mechanisms for stimulating broadly protective immune responses that are cross-reactive against different coronaviruses are not well defined.
Barriers
- SARS-CoV-2 evolves rapidly, leading to the emergence of new viral variants capable of escaping antibody-induced immune protection from vaccination or prior infection.
Gaps
- Immunologic research in the following areas is needed for generating broadly protective coronavirus vaccines:
- Develop a detailed understanding of the human antibody response to SARS-CoV-2 and other coronaviruses, and the interactions between viral responses (Aguilar-Bretones 2023, Pecetta 2022b).
- Identify epitopes (other than the RBD of the S protein) that generate neutralizing humoral immunity and are conserved across different viruses (Cohen J 2021, Crowe 2022, Martinez 2021, Saunders 2021, Walls 2021).
- Identify T-cell epitopes that may provide broader cross-protection against different coronaviruses by stimulating CD4 and CD8 T-cell responses.
- Evaluate whether prior infections to previously or currently circulating coronaviruses, such as SARS-CoV and the common cold coronaviruses, provide cross-protection against heterologous human coronavirus infection (Dangi 2021, Moss 2022).
- Promote understanding of the role of binding but non-neutralizing antibodies versus neutralizing antibodies produced by SARS-CoV-2 vaccines (Poland 2021).
- Improve understanding of receptor-dependent antibody effector functions in developing cross-protection against multiple coronavirus strains (Adams 2022, Mackin 2022).
- Identify mechanisms underlying the induction of broadly protective memory B cells (Wahl 2022).
- Determine the kinetics and magnitude of B-cell response to conserved antigens sufficient to provide broad protection from coronavirus infection, independently or in combination with T-cell responses, for different vaccine platforms (Sette 2022).
- Investigate the potential for immunologic adverse events related to next-generation coronavirus vaccines, such as determining whether increased levels of broadly reactive antibodies exacerbate autoimmune disease by increasing autoreactive antibodies (Labombarde 2022).
Issue: The mechanisms underlying long-term immune responses to coronaviruses require further clarification.
Barriers
- Because initial SARS-CoV-2 infection occurs primarily in epithelial cells on mucosal surfaces, there is limited involvement of systemic immunity, and protective antibody responses are short-lived following infection or injected vaccination (Morens 2022). This is also potentially true for other coronaviruses that cause infection in humans (Belyakov 2009, Karczmarzyk 2022). Furthermore, owing to the short period of mucosal viral replication, natural infection may not be fully controlled by human immune responses, which creates challenges for developing vaccines that are effective and durable (Morens 2023).
- Immune memory responses elicited by vaccines, involving primarily long-lived plasma cells and memory B cells, and possibly memory T cells, are critical for inducing long-term protection, but the mechanisms and determinants of the process are incompletely understood (Gaebler 2021, Inoue 2022, Laidlaw 2022, Siggins 2021, Wahl 2022).
Gaps
- Further research is needed to:
- Understand how prime vaccination, boosting, and immune memory processes interact to generate broadly protective immunity in the context of prior infection, prior vaccinations, or neither. Clarify the relative importance of different components of immune memory for different types of protective immunity, such as against infection, transmission, symptomatic disease, or severe disease.
- Determine the factors that influence duration of antibody and memory B- and T-cell responses following SARS-CoV-2 infection or vaccination (Bhattacharya 2022, Moss 2022, Siggins 2021, Tarke 2022), particularly regarding protection against heterologous strains.
- Determine the length of time that protective immunity from vaccination, either against infection or against the development of severe disease, can be sustained.
- Identify the determinants of longevity for antigen-specific plasma cells in bone marrow and in mucosa-associated lymphoid tissue (Siggins 2021).
- Identify mechanisms that promote persistence of the germinal center following infection and/or vaccination, which is needed to establish immune memory (Laidlaw 2022).
- Define the role of TRM cells and resident memory B cells in the upper and lower respiratory tracts in promoting durable immune protection (Nelson 2021, Sette 2022).
- Determine the kinetics and role of mucosally stimulated antibody and cellular responses.
Issue: The impact of preexisting partial immunity to SARS-CoV-2—whether infection-acquired and vaccine-mediated—on future vaccinations is unknown.
Barriers
- Most of the world’s population has either been infected with SARS-CoV-2 or has been vaccinated against the virus, which complicates research aimed at understanding protective immunologic responses to new vaccines.
- Regional differences likely exist with regard to past exposures to other coronaviruses, which further complicates research efforts.
Gaps
- Further research is needed to:
- Determine levels of baseline immunity to coronaviruses in different populations and assess the impact of preexisting heterosubtypic immunity (e.g., from prior infection with SARS-CoV, MERS-CoV, common cold seasonal coronaviruses, and SARS-CoV-2 variants) on susceptibility to infection and disease from future coronavirus exposures (Bean 2021, Tan 2021, Yu ED 2022).
- Identify mechanisms of imprinting by population characteristics and immune responses to previous exposure to coronavirus vaccines or infection (Mettelman 2022, Pecetta 2022b).
- Determine the effects of repeated booster doses on qualitative and quantitative changes in the humoral and cellular responses to SARS-CoV-2 with different vaccine platform technologies.
- Improve understanding of antigenic imprinting to the S protein, which is important for developing vaccines designed to stimulate immune responses to future SARS-CoV-2 variants and to a broad range of other coronaviruses.
- Better understand glycan masking of antigenic epitopes by preexisting antibodies from vaccination or infection, the role of glycoprotein chemistry in immune imprinting, and its implications for designing broadly protective coronavirus vaccines (Zarnitsyna 2015).
- Clarify the interaction between preexisting immunity and subsequent response to vaccination, including immune kinetics, breadth of protection, durability, and the role of immune memory (Sette 2022).
- Determine how a primed immune system can be reprogrammed or whether preexisting immunity will dominate recall responses (Pecetta 2022b). This includes the need to compare vaccine-induced SARS-CoV-2 immune responses among different populations globally in relation to background immune fitness (e.g., higher inflammation associated with concomitant infections, nutrition, and other factors).
- Clarify the mechanisms of immune durability in the upper and lower respiratory tracts.
Issue: Additional correlates of protection are needed for assessing broadly protective coronavirus vaccines.
- A correlate of protection (CoP) is a measurable biomarker used to reliably predict the level of vaccine efficacy against a clinical outcome, such as vaccine-induced protection against infection, severe disease, or post-acute sequelae of SARS-CoV-2 infection (PASC) (Sherman 2022). The use of CoPs can facilitate down-selecting and vetting of promising broadly protective vaccine candidates for clinical trials and can streamline various aspects of late-stage evaluation, potentially bypassing large-scale field trials by providing a primary end point for provisional or traditional approval of vaccines for specified contexts of use (Karim 2021, Openshaw 2022, Plotkin 2010).
Barriers
- Neutralizing antibody titers have been identified as a CoP for SARS-CoV-2 vaccine efficacy (Gilbert 2022a). CoPs for broadly protective coronavirus vaccine outcomes, however, have yet to be clearly defined and will likely include additional measures of adaptive and innate immune responses (Britto 2022, Gilbert 2022b, Khoury 2021, Morens 2022). The lack of CoPs for broadly protective coronavirus vaccine efficacy may impede advancement of new vaccines through evaluation, regulatory approval, and post-licensure review.
- CoPs against coronavirus infection may be distinct from those against severe disease, and CoPs for mucosal immunity may be distinct from those for systemic immunity (Goldblatt 2022a). It will likely be important to have CoPs that can distinguish between these various situations.
- CoPs for broadly protective vaccines will need to take into account widespread exposures to SARS-CoV-2 antigens via prior vaccination and/or infection.
- CoPs can vary depending on the viral load at exposure, the role of immune memory, individual characteristics such as overall immunostatus, and the method used to detect the CoP (Misra 2022). This creates obstacles for defining the necessary biomarkers to predict coronavirus vaccine efficacy.
- T-cell assays may be important for identifying CoPs for broadly protective coronavirus vaccines; however, they are technically more difficult and costly than serologic assays, and techniques for measuring T cells are currently impractical for clinical trials (Goldblatt 2022a). Furthermore, T cells are often not analyzed from serum samples collected during clinical trials, making it more difficult to draw conclusions about cellular immunity.
- The immune mechanisms of adaptive (humoral and cellular) and innate immune responses that mediate protection against coronavirus infection and disease in different tissues and physiologic compartments are highly complex, which complicates the development of CoPs (Britto 2022, Sui 2021).
- CoPs may vary by host characteristics such as age, gender, preexisting immunity, underlying host genetics, and exposure histories (Sherman 2022, Rodda 2022).
- Different vaccine platforms may have different protective immune mechanisms leading to different CoPs, which can complicate efforts to compare vaccine efficacy among different approaches (Sui 2021).
- Studying the persistence of antibodies following infection is complicated by the lack of standardization of antibody assays, differences in sensitivity and specificity of commercially available assays, and the characteristics of participants studied (Goldblatt 2022a).
Gaps
- To identify CoPs for broadly protective and durable coronavirus vaccines, research is needed to determine the following:
- The kinetics of each relevant type of immune response in the various compartments at different phases of infection, which has implications for the timing of sampling for CoP measurement. Different biomarkers have different durability profiles (e.g., anti-spike neutralizing antibody titers that correlate with short-term protection from symptomatic COVID-19) (Huang 2020).
- Protective thresholds (i.e., a biomarker above a CoP threshold implies a high level of vaccine protection) for different key immune responses in appropriate animal models after infection, vaccination, or both (“hybrid immunity”) (Misra 2022, Suryawanshi 2022, Vardhana 2022). These are important for evaluating vaccine candidates, consistency of production, and updates over time (Goldblatt 2022b, Krammer 2021). Protective thresholds also are needed for different clinical end points (Sherman 2022).
- While protective thresholds provide the most useful CoPs, the goal to reliably predict vaccine efficacy in some clinical context of use might be satisfied with a CoP that uses the whole distribution of the immunologic biomarker or uses other features besides a threshold cutoff such as geometric mean. This requires a statistical algorithm for predicting vaccine efficacy based on measuring the immunologic biomarker from a sample of vaccine recipients (and possibly also from another group of comparator vaccine recipients for comparison), where this algorithm may or may not make use of a threshold cutoff for the CoP.
- There is a potential need for multiple biomarkers to increase the reliability of measurements for different intended outcomes (Jang 2020, Misra 2022, Plotkin 2020). Key components relevant to durable and broadly protective immune responses include neutralizing antibodies, memory B cells, Fc effector antibodies, and CD4 and CD8 T cells (Goldblatt 2022a, Kaplonek 2022, McGrath 2022).
- More information is needed on biomarkers for various patterns of hybrid immunity (based on prior infection, vaccination, interval between vaccine doses, vaccine platform, etc.) to SARS-CoV-2 (Rodda 2022).
- Reliable CoPs will be needed for different coronavirus vaccine constructs, based on different antigens (potentially strain-specific and broadly protective antigens), different vaccine platforms, and different modes of administration, in conjunction with the development of appropriate animal models and the establishment of regulatory pathways for their review (Krammer 2022).
- Even though neutralizing antibodies to the S protein appear to be a reasonable CoP for SARS-CoV-2 vaccines, the titers that correlate with protection in populations with different histories of exposure to SARS-CoV-2 viruses need to be determined (Simon 2022).
- Efforts are needed to develop mechanistic CoPs, which require a deeper understanding of innate and adaptive (humoral and cellular) immune responses (Britto 2022).
- Expanded use of advanced systems-biology tools, such as proteomics and systems serology, along with machine learning, is needed to support the development of mechanistic CoPs (Britto 2022).
- Research into CoPs for additional coronavirus vaccines will require the availability of the necessary reagents and virus stocks.
- Defining and harmonizing clinical or efficacy end points is necessary for determining and comparing CoPs for different vaccines (Sherman 2022).
- Standardized, validated high-throughput assays for T-cell responses are needed to advance CoP development and facilitate their use in clinical trials (Goldblatt 2022a, Huang 2020, McGrath 2022, Misra 2022, Pecetta 2022b, Vardhana 2022).
- Innovation is needed to scale up T-cell assays by simplifying sample collection and storage, and standardizing data collection and laboratory methods.
- A central database that includes potential CoPs for current vaccines could be useful to assess multiple variables as CoPs and to test if a CoP identified in one trial is valid in other trials (Karim 2021). Depending on the use or immunobridging application of a CoP, the CoP may differ and the means to validate the CoP may differ; accordingly, the central database needs to include adequate meta-data to support the ability of data analyses to meet objectives.
- Once one or more CoPs are identified, standardized assays are needed for that CoP to ensure comparability between different vaccine platforms, modes of administration, and conditions of use (Sherman 2022, Krammer 2022).
Strategic Goals and Aligned Milestones for Topic 2: Immunology and Immune Correlates of Protection
Strategic Goal 2.1: Ensure that clinical samples and immunoassays are available to the research community for improving understanding of the mechanisms of mucosal and systemic immunity related to SARS-CoV-2 infection. |
Milestone 2.1.a (High Priority): By 2023, develop a centralized or virtual biorepository, and an associated governance structure, to use pre-COVID-19 pandemic clinical samples, including mucosal (e.g., nasal lavage and saliva) and serologic samples that are currently available from a range of research laboratories, potentially by tapping into existing biobanks. |
Milestone 2.1.b: By 2023, create a plan for assay development aimed at generating assays to answer the key immunologic mechanistic questions related to SARS-CoV-2 that the biorepository samples can address. |
Milestone 2.1.c (High Priority): By 2024, establish and fund a centralized or virtual biorepository involving a new cohort of subjects from multiple regions of the world, to include those with a history of SARS-CoV-2 infection, for obtaining high-impact (e.g., mucosal, bronchoalveolar lavage, serologic, bone marrow) and appropriately collected and timed clinical samples. |
Milestone 2.1.d (High Priority): By 2024, develop new immunologic assays for SARS-CoV-2 research, as outlined in the plan identified in Milestone 2.1.b, and ensure that such assays are appropriately harmonized, standardized, and reproducible. |
Milestone 2.1.e: By 2027, develop immunologic assays for a broader range of coronaviruses that are harmonized, standardized, and reproducible. |
Strategic Goal 2.2: Define mechanisms of mucosal and systemic immunity relevant to SARS-CoV-2 infection and the development of broadly protective coronavirus vaccines. |
Milestone 2.2.a: By 2025, define the initial humoral mechanisms of protection at the mucosal barrier for SARS-CoV-2 infection. |
Milestone 2.2.b: By 2026, define the initial cellular mechanisms of protection at the mucosal barrier for SARS-CoV-2 infection. |
Milestone 2.2.c: By 2026, determine the relative roles of mucosal (in the upper and lower respiratory tracts) versus systemic humoral immunity in protecting against coronavirus infection and limiting the potential for virus transmission (Mettelman 2022, Poland 2021). |
Milestone 2.2.d (High Priority): By 2027, determine mucosal biomarkers, including systemic surrogates of mucosal immunity (Matuchansky 2021), that are predictive of mucosal immune protection against SARS-CoV-2 infection. |
Milestone 2.2.e: By 2027, develop a mucosal immunity “atlas”—a comprehensive characterization of mucosal immunity—to collect and organize information on innate and adaptive coronavirus mucosal immunity that maps responses in different anatomic compartments (i.e., upper versus lower respiratory tract) across different age-groups and geographies. |
Strategic Goal 2.3: Clarify mechanisms for stimulating broadly protective mucosal and systemic immune responses that are cross-reactive for different coronaviruses. |
Milestone 2.3.a (High Priority): By 2024, identify epitopes other than the RBD area of the S protein that generate protective humoral immunity and are conserved across virus types (Cohen J 2021, Crowe 2022, Martinez 2021, Saunders 2021, Walls 2021). |
Milestone 2.3.b: By 2025, identify broadly protective antibodies against the conserved S2 region of the SARS-CoV-2 spike protein, which may be critical for developing broadly protective coronavirus vaccines (Zhou 2022). |
Milestone 2.3.c: By 2025, identify mechanisms underlying the induction of broadly protective antibodies, such as via production and recall of long-lived memory B cells that recognize conserved epitopes in SARS-CoV-2 viruses (Qi 2022). |
Milestone 2.3.d: By 2026, identify T-cell epitopes for non-spike proteins that may provide broad cross-protection against different coronaviruses by stimulating CD4 and CD8 T-cell responses. |
Strategic Goal 2.4: Understand the mechanisms of durability of immune protection from SARS-CoV-2 and other coronaviruses. |
Milestone 2.4.a (High Priority): By 2024, determine initial factors that influence duration of antibody and memory B- and T-cell responses following SARS-CoV-2 infection or vaccination, such as persistence of the germinal center (Bhattacharya 2022, Moss 2022, Siggins 2021, Tarke 2022). |
Milestone 2.4.b: By 2029, identify the determinants of longevity for antigen-specific plasma cells in bone marrow and in mucosa-associated lymphoid tissue (Siggins 2021). |
Strategic Goal 2.5: Improve understanding of the impact of preexisting immunity—both infection-acquired and vaccine-mediated—on immune responses to future circulating coronaviruses. |
Milestone 2.5.a: By 2025, clarify the interaction between preexisting immunity to coronaviruses and subsequent response to vaccination, such as by evaluating immune kinetics, breadth of protection, the role of epitope masking, and the role of immune memory (Sette 2022). |
Milestone 2.5.b: By 2026, ensure that longitudinal cohort studies are implemented to determine levels of baseline immunity to coronaviruses in geographically diverse populations and assess the impact of preexisting heterotypic immunity—as from prior infection with SARS-CoV, MERS-CoV, common cold coronaviruses, and SARS-CoV-2 variants—on susceptibility to infection and disease from future coronavirus exposures (Bean 2021, Tan 2021, Yu ED 2022). |
Strategic Goal 2.6: Identify mechanistic correlates of protection for immunity generated by SARS-CoV-2 vaccines and broadly protective coronavirus vaccines. |
Milestone 2.6.a: By 2023, create a central database, primarily from observational studies, that includes potential CoPs for current SARS-CoV-2 vaccines to allow different investigators to assess multiple or alternative CoPs (Karim 2021), with new information being added as it becomes available. |
Milestone 2.6.b: By 2025, define and harmonize the clinical or efficacy end points (e.g., mild vs. severe disease) for determining and comparing CoPs for different SARS-CoV-2 vaccines (Sherman 2022). (See Vaccinology.) |
Milestone 2.6.c (High Priority): By 2026, identify statistically validated CoPs for predicting the efficacy of SARS-CoV-2 vaccines based on key immune responses that correlate with specific clinical end points and that are applicable to viral variants with different characteristics. |
Milestone 2.6.d (High Priority): By 2027, determine one or more CoPs for mucosal vaccines against SARS-CoV-2 infection. |
Milestone 2.6.e: By 2027, determine whether assays or surrogate markers for T-cell responses could serve as CoPs for current or new broadly protective coronavirus vaccines. |
Milestone 2.6.f: By 2027, conduct studies in animal models to identify CoPs for evaluating broadly protective coronavirus vaccines. |
Milestone 2.6.g: By 2028, determine whether multiple biomarkers are needed to increase the performance of a CoP for predicting vaccine efficacy of SARS-CoV-2 vaccines or broadly protective coronavirus vaccines (Jang 2020, Misra 2022, Plotkin 2020), such as neutralizing antibodies, memory B cells, Fc effector antibodies, and CD4 and CD8 T cells (Goldblatt 2022a, Kaplonek 2022, McGrath 2022). |
Milestone 2.6.h: By 2028, ensure that one or more CoPs for assessing broadly protective coronavirus vaccines are validated. |
Milestone 2.6.i: By 2028, standardize and develop mechanisms to harmonize CoP assays to facilitate comparability among different vaccine platforms and modes of administration (Sherman 2022, Krammer 2022). |
Additional Research Priorities
- Continue to study how innate immunity influences adaptive (B-cell and T-cell) immune responses to SARS-CoV-2 infection (Sette 2021, Tomalka 2022), particularly among different age-groups and global regions or populations, including people who are immunocompromised.
- Develop a detailed understanding of the human antibody response to SARS-CoV-2 and other coronaviruses (Pecetta 2022b).
- Determine how SARS-CoV-2 variants (and potentially other coronaviruses) evade immune responses, including antibody and T-cell responses.
- Determine the potential for improving breadth of protection against coronaviruses by stimulating innate “trained” immunity (Mettelman 2022, Ziogas 2022).
- Determine the role and mechanisms of adjuvants in mediating interactions between innate and adaptive immune responses (Carmen 2021, Lee 2022), such as those that drive a breadth of response via CD4 T-cell activation (Joyce 2022).
- Clarify the role of memory T cells in reducing disease severity (Kent 2022, Moss 2022, Zheng 2022).
- Continue to clarify the role of TRM cells and resident memory B cells in the upper and lower respiratory tracts in promoting durable immune protection (Nelson 2021, Sette 2022).
- Identify mechanisms of imprinting by population characteristics and immune responses to previous exposure to coronavirus vaccines or infection (Mettelman 2022, Pecetta 2022b).
- Determine how preexisting immunity affects recall responses and how a primed immune system can be induced to generate broadly protective immune responses to divergent coronaviruses (Pecetta 2022b).
- Continue to assess the role of different immune compartments and components of adaptive immunity to SARS-CoV-2 virus infection and vaccination or to other coronaviruses, with a focus on the specific functions and kinetics of the three key components of the adaptive immune response (Moss 2022, Sette 2021, Sette 2022):
- B cells, the source of neutralizing antibodies
- CD4 T cells, which produce helper T cells, Th1 cells, and T follicular helper cells
- CD8 T cells, which kill infected cells
- Clarify the role in immune protection of binding but not neutralizing antibodies produced by coronavirus vaccines (Poland 2021).
- Determine the kinetics and magnitude of B-cell responses to conserved antigens sufficient to provide broad protection from coronavirus infection, independently or in combination with T-cell responses, for different vaccine platforms.
- Continue to expand the use of advanced systems-biology tools, such as proteomics and systems serology, along with machine learning, to support immunologic research germane to broadly protective coronavirus vaccines.
- Continue to assess the potential for immune-mediated enhanced disease following vaccination in the context of future SARS-Co-V-2 variants (Bigay 2022).
- Determine whether increased levels of broadly reactive antibodies exacerbate autoimmune disease by increasing autoreactive antibodies (Labombarde 2022).
- Determine the immunopathologic mechanism of SARS-CoV-2 vaccine-induced myopericarditis.
- Determine the role for activating multiple arms of the immunity system—including T cells, non-neutralizing antibodies, neutralizing antibodies to conserved epitopes, innate immune responses, and mucosal immunity—in eliciting broadly protective immunity (Hauser 2022).
- Continue to employ innovative techniques to scale up T-cell assays by simplifying sample collection and handling, and standardizing data collection and laboratory methods.
- Consider studies with experimental manipulation of immune markers that enable more direct assessment of mechanistic CoPs, including vaccine challenge studies, monoclonal antibody challenge studies, and field trials of monoclonal antibodies for prevention.
- Determine the processes by which immune dysregulation may contribute to severe COVID-19 disease following infection and the implications for developing next-generation coronavirus vaccines.
- Continue to assess the evolution of the human B-cell repertoire and antibody responses after SARS-CoV-2 infection and immunization to determine the underlying parameters that contribute to broadening of immune responses (Pecetta 2022b).
This section addresses priority areas in the topic of vaccinology needed to advance R&D for broadly protective coronavirus vaccines, such as development of preferred product characteristics. Advancements in new technologies, research methods, platforms, adjuvants and innovative delivery methods will accelerate the development and distribution of broadly protective vaccines. Long-term commitment and investment will be essential to advance new candidate vaccines through the full pipeline from discovery to regulatory approvals.
Issue: A set of preferred product characteristics (PPCs) for broadly protective coronavirus vaccines that has been widely vetted and agreed upon by key stakeholders is needed to inform vaccine R&D.
Barriers
- Since the next coronavirus threat is unknown in terms of timing and source of emergence, transmissibility, morbidity, mortality, and clinical presentation, typical risk-benefit calculations for broadly protective coronavirus vaccines are not possible.
- Development of preferred product characteristics (PPCs) is complicated by the potential need for different product characteristics for vaccines that target different viruses, are designed for different at-risk groups or ages, or have different indications (e.g., transmission blocking vs. reducing morbidity and mortality).
Gaps
- Both PPCs and target product profiles (TPPs) outline the preferential attributes for vaccines under development. PPCs address early-stage research and are intended to promote innovation by providing broad guidance on the development of new products or the improvement of existing products. PPCs are not specific to any particular product and provide general guidance on attributes related to vaccine indications, target populations, and vaccine use cases (WHO IVB). TPPs are typically more specific and provide parameters that can inform R&D targets for funders and developers; they are often used as planning tools for entities that are aiming for regulatory approval or WHO prequalification (WHO IVB). Important efforts have gone into the development of TPPs for broadly protective coronavirus vaccines. A set of PPCs is also necessary to provide overall guidance to the research community and to industry regarding key characteristics for such vaccines. Issues to consider when developing PPCs include:
- Guidance on the short-term versus long-term goals will help bring the needed vaccines to market most efficiently (e.g., SARS-CoV-2 variant-proof vaccines vs. vaccines that protect against multiple coronavirus species).
- Consensus on vaccine efficacy end points, such as blocking infection, interrupting transmission, and/or mitigating morbidity and mortality (perhaps to include long COVID/PASC) will help prioritize efforts in bringing next-generation broadly protective vaccines to market.
- Clarity on how durability can be measured and against which outcomes, factors affecting durability, whether or not vaccines can be made more durable and for how long, and defining realistic expectations for vaccine durability.
- Consensus on how broadly protective coronavirus vaccines will be used (e.g., proactively as part of routine vaccination programs vs. reactively as part of a pandemic or epidemic response strategy). This will inform what is envisioned as the end products, may influence the regulatory pathway, and will determine the markets for different approaches.
- Emphasis is also needed on the importance of developing vaccines that are suitable for use globally, including use in remote and low-resource settings.
- Many of the innovative immunogen designs for broadly protective coronavirus vaccines have extremely complex manufacturing processes, which should be taken into consideration when deciding which vaccine candidates should advance through the development pipeline, as this will influence the ability to manufacture such vaccines in LMICs or other low-resource settings.
- Up-front discussions are needed on how to simplify distribution, administration schedules (e.g., spacing, number of doses, mode), and stability (e.g., cold chain and storage requirements) without sacrificing vaccine safety or effectiveness, to facilitate equity in vaccine access (Rees 2022a).
- Since developing broadly protective coronavirus vaccines will be difficult, especially considering the many issues outlined above, it is important to define a minimally acceptable TPP or set of PPCs that focus on broad protection as a starting point.
Issue: Broadly protective coronavirus vaccine candidates will need to provide protection against a range of existing and novel coronaviruses while maintaining vaccine safety.
Barriers
- Many novel vaccine technologies and approaches for eliciting broad protection against coronaviruses are under investigation, but additional ongoing resources and investments will be needed to incentivize and move new vaccine candidates through the development pipeline, particularly into clinical trials where safety and efficacy can be adequately assessed (although even with relatively large clinical trials, post-marketing surveillance for rare adverse events is also essential).
- Selection of antigens to optimize broad immunogenicity and cross-reactivity is challenging owing to the phylogenetic diversity of coronaviruses, antigenic breadth within coronaviruses, and limited understanding of conserved B- and T-cell epitopes across different coronavirus subgroups (Baric 2022, Pack 2022). (See Virology Applicable to Vaccine R&D.)
- Multiple scientific, methodologic, and regulatory challenges exist for development of vaccines against pathogens that have not yet emerged, or for vaccines aimed at addressing future explosive outbreaks.
Gaps
- Researchers are studying a variety of antigen presentation strategies for eliciting broad protection, such as focusing on highly conserved viral regions and using multiplexed chimera or nanoparticle vaccine technologies (Chiu 2021, Martinez 2021, Saunders 2021, Walls 2021, Joyce 2022). At this time, it remains unclear as to which of these approaches are the most efficacious for broadly protective coronavirus vaccines.
- Further research into immunogenic antigens other than the S protein may identify novel vaccine targets that could be more broadly protective, such as the nucleocapsid, membrane, or envelope proteins (Poland 2020, Soraci 2022).
- It is unclear which vaccine platforms will induce the broadest and most durable protection. A number of platforms are under investigation, such as live-attenuated virus vaccines, whole inactivated virus vaccines, viral-vectored vaccines, recombinant protein subunit vaccines, virus-like particle and nanoparticle vaccines, and nucleic acid (deoxyribonucleic acid [DNA] or RNA) vaccines (Begum 2021, Li 2020, Sung 2021, WHO 2023).
- Additional efforts are needed to track and analyze the vaccine landscape for broadly protective coronavirus vaccine candidates and identify the paths that vaccine developers in industry, academia, and government laboratories can take—as well as to identify the potential pitfalls—to stimulate and maintain productive vaccine R&D.
- Many of the vaccine platforms under evaluation for generating broad protection are relatively new and do not have long safety records. For example, post-marketing surveillance identified myocarditis as an adverse event associated with messenger RNA (mRNA) vaccines (Bots 2022), and thrombotic events have been identified following use of adenovirus-vectored vaccines (Mani 2022). As additional novel approaches for broadly protective coronavirus vaccines are investigated, ongoing efforts are needed to promote adequate evaluations of vaccine safety both along the pre-approval pipeline and in post-marketing adverse event surveillance around the globe (Rizk 2021).
- Further research is needed into antigenic imprinting and heterologous prime-boost vaccination strategies for generating broad protection against multiple coronavirus strains (Shepherd 2022, Tan 2021). For example, different vaccination approaches, such as immunization schedules with multiple boosters, differing schedules, and the use of different vaccine platforms in a prime-boost heterologous strategy, may produce a more effective response than modifications to antigens or adjuvants alone (Shepherd 2022). This should be assessed across different geographic regions.
- Understanding SARS-CoV-2 mutations and evolution, which is necessary for vaccine development, can be enhanced by an expansion of whole-genome sequencing and genomic databases, bioinformatics approaches, structure-based rational immunogen design, antigenic mapping, and computational analyses assisted by machine learning (Soraci 2022, Pack 2022). (See Virology Applicable to Vaccine R&D.)
- The impact of immune imprinting and preexisting partial immunity to SARS-CoV-2 (infection-acquired and vaccine-mediated) on future vaccinations is unknown. (See Immunology and Immune Correlates of Protection.)
- Immunologic assays are needed to evaluate broadly protective coronavirus vaccines and determine such factors as the impact of preexisting immunity on vaccine performance, vaccine performance in naïve or vulnerable populations, and the breadth and durability of vaccine protection [Baric 2022, Goldblatt 2022a, Vardhana 2022]). Such assays are also important for identifying CoPs, which play an important role in vaccine evaluation. (See Immunology and Immune Correlates of Protection.)
Issue: Candidate vaccines need to elicit durable protection.
Barriers
- Durability of protection is not easily assessed in humans or in animal models, given the lack of immune CoPs against infection and particularly against severe disease (Altmann 2022), and the lack of early immune signatures for durable immunity. Identifying CoPs requires models that produce immune responses with differential durability (See Animal and Human Infection Models.)
- Determining how best to assess vaccine durability in preclinical development remains a major scientific challenge.
- Sustained protection against infection and disease relies on both neutralizing responses and non-neutralizing responses, such as T cells, memory B cells, and Fc dependent humoral responses (Krause 2022, Hsieh 2021). Defining the roles of these different systemic and mucosal responses in promoting durability, particularly across different coronaviruses, requires further investigation. (See Immunology and Immune Correlates of Protection.) Additionally, immune markers for all of these responses are not readily available.
- Clinical trials may require 1 to 2 years or multiple seasons of follow-up to determine vaccine durability, which adds cost and complexity to research efforts and raises issues of feasibility (Hodgson 2021).
- Repeated boosting with additional doses of existing vaccines or with slightly modified vaccines may limit the ability to study novel vaccines that elicit more broadly protective responses (Pack 2022).
- Vaccines are often licensed and used to respond rapidly to an outbreak before a detailed understanding of vaccine durability is available.
Gaps
- The length and type of protection (e.g., from hospitalization, death, reinfection, and/or transmission) expected from durably protective vaccines are not well-defined (Pack 2022).
- More information is needed regarding the durability afforded by different vaccine platforms.
- More information is needed on the impact of different types of adapted vaccines on protection from disease and hospitalization.
- Adjuvants and carefully designed immunization schedules that involve periodic boosting may or may not be needed to stimulate effective and long-term protection in a primed population (Altmann 2022, Pack 2022).
Issue: Further optimization of coronavirus vaccines is needed to improve access to future vaccines within and across different populations.
Barriers
- Stimulating mucosal immunity may be important for promoting breadth and durability of protection (Bhattacharya 2022) and may also be necessary to prevent viral entry into mucosal cells, which will prevent infection and decrease the potential for asymptomatic transmission of coronaviruses (Soraci 2022). Current injectable coronavirus vaccines do not appear to significantly stimulate adequate mucosal immunity (Azzi 2022, Collier 2022).
- Some technologies under investigation, such as live-attenuated virus vaccines, may not be appropriate for those who are pregnant, the elderly, or others with compromised immune systems (Ansariniya 2021, Soraci 2022).
- Route of administration for future coronavirus vaccines could include existing or novel approaches to vaccine administration (intramuscular, transdermal, oral, inhalation or nasal); experience with alternative routes of administration is limited.
Gaps
- Additional efforts are needed in the following areas to optimize future coronavirus vaccines:
- Research into vaccines that stimulate mucosal immunity (including immunoglobulin A [IgA] antibodies, local mucosal immunoglobulin G [IgG] production, and cytotoxic T lymphocyte activation) and will likely be administered intranasally or orally. An important issue for mucosal vaccines is the need to establish a CoP for mucosal immunity. (See Immunology and Immune Correlates of Protection.)
- Improvements in vaccine thermal stability to address cold-chain issues that may limit access to certain vaccine platforms in remote or low-resource settings (Soraci 2022).
- Strategies to increase vaccine immunogenicity among people who are immunocompromised, frail, or elderly (Sung 2021).
- Research to determine the role of different adjuvants for improving immunogenicity of next-generation coronavirus vaccines, including the design, development, and selection of the most potent adjuvants for different vaccine platforms (Pack 2022).
- Improved understanding of issues associated with maternal vaccination.
Issue: Clinical trial design or other alternative approaches for demonstrating efficacy, non-inferiority, or superiority is complicated for broadly protective coronavirus vaccines.
Barriers
- The target virus (or viruses) must be circulating in humans to perform the gold standard randomized controlled trial (RCT) for vaccine efficacy (Hodgson 2021). RCTs that assess the efficacy of a vaccine across the full breadth of its protection may not be possible for viruses or variants that are not yet circulating in the human population, although it may be possible to conduct RCTs to determine if a broadly protective vaccine is superior or non-inferior to approved vaccines against circulating SARS-CoV-2 strains.
- Broad protection and cross-reactive immunity will need to be assessed in naïve, previously vaccinated, and previously infected people, which adds complexity to future research (Pecetta 2022b).
- For SARS-CoV-2 variants, vaccine efficacy assessed during clinical trials is difficult to extrapolate, because results will depend on which strains are circulating in the area at the time of the trial (Pecetta 2022b).
- Differences in vaccine efficacy are likely to be observed in different geographic locations, not just because of differences in the circulating strains or prevalence of infection or vaccination, but also because of health factors such as demographics, poverty, malnutrition, access to high-level medical care, and prevalence of comorbidities, including HIV (Hodgson 2021).
Gaps
- The absence of standardized or harmonized clinical trial end points, outcomes of interest, and assays for the evaluation of the human immune response makes interpretation and comparison of clinical trial data difficult (Pecetta 2022b).
- A framework, such as a platform trial, is needed to assess mucosal vaccines with clinical end points, such as infection, disease, and transmission.
- The research and regulatory communities will need to establish how to best assess the efficacy of broadly protective coronavirus vaccines in light of preexisting immunity, either from infection or vaccination (Rees 2022a). Additionally, it is unclear what regulators will require for demonstrating breadth of protection.
- Researchers may need to use one or more CoPs or well-characterized immune markers as surrogate end points for assessing vaccine efficacy in the absence of circulating virus (Krause 2022); however, more efforts are needed to define them. (See Immunology and Immune Correlates of Protection.)
- Owing to challenges with conducting clinical trials for broadly protective coronavirus vaccines, alternative approaches for assessing vaccine efficacy may be necessary. For example, some have proposed an alternative framework that involves comparing a new vaccine to an approved vaccine. Examples of issues regarding using this framework include (Krause 2022):
- The selection of comparator vaccines will rely on availability and a solid knowledge base for existing vaccines; therefore, researchers need to ensure that adequate information for the comparator vaccine is available.
- The framework requires the ability to make direct or indirect comparisons of immune responses induced by the new and the comparator vaccine; therefore, a thorough understanding of the immune responses for each vaccine will be necessary.
- If neutralizing immune responses are used for immunobridging, they will need to be predictive of other overall protective responses. Data validating this concept will be needed.
- More research is needed regarding whether or not vaccines involving different platforms can be compared to each other.
- Other approaches for assessing efficacy exclusive of clinical trials include animal studies with further immunobridging to human populations or human infection studies. Additional efforts are needed to clarify how these alternative strategies can be used to assess vaccine efficacy, particularly for determining breadth of protection. (See Animal and Human Infections Models.)
Issue: The regulatory pathway demonstrating efficacy, non-inferiority, or superiority is particularly complicated for coronavirus vaccines designed to be broadly protective.
Barriers
- It will be challenging to do more than lay out the regulatory strategies for approval of any broadly protective coronavirus vaccine until the characteristics of the viruses, the characteristics of the vaccine, and the potential indications for the vaccine’s use are known.
- Accelerated approval pathways or emergency use authorization may be options for authorization in the case of a marked increase in the sense of urgency, which is what occurred early in the COVID-19 pandemic. Use of these pathways, however, depends on a high level of public health risk (Beasley 2016, WHO 2020b). Furthermore, opportunities for emergency use authorization and expedited licensing procedures for coronavirus vaccines may be more limited in the future (Branswell 2022), unless new SARS-CoV-2 variants emerge that create a renewed sense of urgency.
- Good clinical practice (GCP), good manufacturing practice (GMP), and good laboratory practice (GLP) form the foundation for regulatory compliance. Vaccines are approved in the country of manufacture by a national regulatory authority (NRA), yet not all NRAs are stringent with their GMPs, and not all countries have the capacity within their NRAs to ensure GMP, which could lead to lapses in regulatory compliance for coronavirus vaccines (Brüssow 2021).
- Regulatory issues focused on specific products cannot be readily discussed multilaterally among NRAs and instead are limited to bilateral discussions among NRAs that have non-disclosure agreements in place (Farley 2022, Cavaleri 2022).
- Broadly protective coronavirus vaccines will likely need to show protection not only against coronaviruses that are circulating in the human population but also against viruses that are not circulating, which creates additional challenges for regulatory approval and for determining what information can be included in product labeling.
Gaps
- There are three potential pathways in the United States for regulatory approval of vaccines: the traditional pathway that relies on efficacy data from RCTs, the accelerated approval pathway that determines efficacy in field trials based on a surrogate marker, and approval via the US Food and Drug Administration’s (US FDA’s) Animal Rule that involves efficacy data generated from animal studies with immunobridging to humans if the other pathways are not feasible and the requirements of the Animal Rule can be met (US FDA 2011, US FDA 2022). Regulatory authorities in other countries have similar pathways, although they generally lack an animal rule option; however, animal data could still play a pivotal role in the benefit-risk assessment. Additionally, data from human infection studies could be used to assess vaccines and quickly determine whether a vaccine is likely to produce clinical benefit, and in some instances may be used to assess efficacy (US FDA 2011). Additionally, such studies could also identify a surrogate marker or an immune CoP for a given vaccine that could be used in larger field trials to further assess efficacy, which is being considered for other pathogens (Deming 2020, Giersing 2019). Animal and human infection studies could be of value for vaccine approval if clinical efficacy trials cannot be done, such as if SARS-CoV-2 variants are no longer circulating in the population. At this time it remains unclear, however, as to what information or pathways will be acceptable for regulatory approval for broadly protective coronavirus vaccines, other than traditional approval.
- Vaccine developers need guidance regarding what information regulatory agencies will potentially accept for defining efficacy of variant-proof SARS-CoV-2 vaccines or broadly protective coronavirus vaccines.
Strategic Goals and Aligned Milestones for Topic 3: Vaccinology
Strategic Goal 3.1: Define goals for broadly protective coronavirus vaccines by establishing a widely agreed upon and vetted set of PPCs and determine use cases for such vaccines. |
Milestone 3.1.a (High Priority): By 2023, building on existing TPPs, develop a broadly agreed upon and internationally vetted (e.g., through a process involving an international multilateral organization such as the WHO) set of PPCs to identify key product characteristics, including optimal and critical minimal criteria. These could follow a tiered approach, with an initial focus on variant-proof SARS-CoV-2 vaccines, then moving to other, more broadly protective tiers. |
Milestone 3.1.b: By 2024, develop initial use cases for broadly protective coronavirus vaccines, defining how, where, and under what circumstances such vaccines would be used, such as defining target populations, addressing cold-chain and vaccine stability considerations, and exploring equitable access in resource-constrained settings. Following initial development, the use cases and PPCs may need to be modified over time through an iterative process. |
Strategic Goal 3.2. Leverage new technologies or new approaches to create effective, durable, and safe vaccines that offer broad protection across different coronaviruses. |
Milestone 3.2.a: By 2023, define a set of principles that can be used by funders and developers to down-select vaccine candidates for further evaluation. This set of principles should take into consideration issues such as complexity of manufacturing, key challenges with vaccine distribution and use, and the end goals for using different vaccines. All of these issues influence the ability of vaccines to be manufactured and used in different global regions, including in LMICs. |
Milestone 3.2.b (High Priority): By 2023, publish the findings of a workshop on SARS-CoV-2 mucosal vaccines to identify gaps in mucosal approaches for vaccine development. |
Milestone 3.2.c: By 2024, advance a strategy or mechanism to promote collaboration among researchers and developers aimed at combining technologies to expand breadth of coronavirus vaccine coverage, such as assessing combinations of vaccines or different antigens in animal models or early clinical trials, or assessing prime-boost combinations of different approved or candidate vaccines. |
Milestone 3.2.d (High Priority): By 2024, develop and make available to researchers an initial repository of coronaviruses (as available), pseudoviruses (if they can be made), and antigens. The repository could be developed in a tiered fashion, with an initial focus on the highest-risk viruses and then adding additional viruses over time. |
Milestone 3.2.e: By 2024, create a comprehensive landscape of broadly protective coronavirus vaccine candidates in preclinical and clinical development and create a mechanism to update and analyze the landscape, including identifying key factors underlying successful R&D efforts as well as persistent challenges and obstacles. |
Milestone 3.2.f: By 2024, conduct an analysis of existing adjuvants and create a repository of available adjuvants to ensure they are accessible and available to vaccine R&D researchers. |
Milestone 3.2.g: By 2024, evaluate the pathophysiology of rare adverse events associated with existing SARS-CoV-2 vaccines, and determine the potential risk of similar adverse events with the most promising broadly protective approaches. |
Milestone 3.2.h: By 2025, determine, primarily through preclinical studies, if any adjuvants can substantially improve vaccine efficacy, breadth, or durability for SARS-CoV-2 variants and other coronaviruses without compromising safety. |
Milestone 3.2.i (High Priority): By 2027, further clarify, through clinical studies, if alternative routes of administration, including intranasal, transdermal, inhaled, and oral vaccines, can enhance mucosal immunity and protect against disease and transmission. |
Strategic Goal 3.3. Establish principles for conducting clinical trials that allow for comparisons between vaccines. |
Milestone 3.3.a: By 2024, develop a set of harmonized clinical end points—such as infection, severe disease, death, and potentially long COVID/PASC— and immunologic end points that can be used in vaccine efficacy studies for broadly protective coronavirus vaccines to demonstrate protection against SARS-CoV-2 variants and “common cold” coronaviruses. |
Milestone 3.3.b: By 2025, develop a process involving cross-cutting experts and regulators for rapidly identifying and agreeing on standardized clinical and/or immunologic end points that can be used to capture vaccine efficacy quickly after the emergence of a novel coronavirus. |
Milestone 3.3.c: By 2025, based on outcomes of the previous milestones, status of scientific knowledge, and circulating viruses at the time, develop and disseminate an international concept protocol that includes principles for clinical trials to allow for comparisons between broadly protective vaccine candidates and comparator vaccines. |
Strategic Goal 3.4. Build a foundation for regulatory evaluation of future coronavirus vaccines. |
Milestone 3.4.a: By 2023, initiate annual meetings between the scientific community, regulatory authorities, and vaccine developers to share the latest immunology, virology, vaccinology, and regulatory science advances and challenges to assist in building a foundation for regulatory evaluation of new coronavirus vaccines. |
Milestone 3.4.b (High Priority): By 2023, develop a set of principles to inform regulatory evaluation of new coronavirus vaccines that outlines what information is required to demonstrate the need for and added value of variant-proof SARS-CoV-2 vaccines and to provide confidence in vaccine efficacy, particularly compared to approved vaccines. This set of principles would be less specific than regulatory guidance, since many details will still be unresolved, but such principles could be a valuable starting point for clarifying regulatory evaluation for such vaccines. |
Milestone 3.4.c (High Priority): By 2025 and building on Milestone 3.4.b, develop a set of principles for regulatory evaluation of future more broadly protective coronavirus vaccines that: (1) follows a tiered or stepwise approach (such as starting with predicting efficacy against sarbecoviruses other than SARS-CoV-2, then to merbecoviruses, and then to additional coronaviruses of concern as necessary); (2) takes into consideration the various mechanisms of protection that different vaccines may employ, which may inform the potential breadth of protection for a given vaccine construct; (3) clarifies what a “broadly protective coronavirus vaccine” means from a regulatory perspective and how breadth of protection is communicated in the associated product information; (4) identifies approaches for predicting protection provided by new vaccines (i.e., predicting potential clinical benefit) against coronaviruses that are not circulating in the human population; (5) defines the potential roles and limitations of tools such as animal studies, human infection studies, and immunobridging for predicting the breadth of protection for new vaccines; and (6) clarifies potential regulatory pathways for new coronavirus vaccines. |
Strategic Goal 3.5. Facilitate the development of vaccine candidates with characteristics that meet global needs. |
Milestone 3.5.a: By 2023, begin to advance the involvement of LMICs in clinical development programs, so that clinical trials of broadly protective coronavirus vaccines include LMIC settings. |
Milestone 3.5.b: By 2026, support the development of broadly protective coronavirus vaccines that can be made with less-complex manufacturing systems, to ensure the potential to manufacture such vaccines in more regions, which could lead to more equitable distribution. |
Milestone 3.5.c (High Priority): By 2027, support the development of coronavirus vaccine technologies that are suitable for broad access and global distribution, such as cold-chain–independent technologies; are scalable; and can be produced affordably. |
Additional Research Priorities
- Continue to expand the use of whole-genome sequencing and genomic databases, bioinformatics approaches, structural vaccinology, and computational analyses to improve vaccine design (Soraci 2021, Pack 2022).
- Evaluate, on an ongoing basis, the potential for antigenic drift among conserved epitopes under immune pressure, as when epitopes are used as an antigenic target for broadly protective vaccines.
- Continue to evaluate the potential for VAED as new broadly protective coronavirus vaccines are developed (Gartlan 2022).
- Continue to build capacity and collaboration among NRAs worldwide, including joint reviews of clinical trials and licensure applications, agreement on global standards for licensure of protective coronavirus vaccines, and development of processes to allow for timely and streamlined licensure in geographically diverse areas for global vaccine roll out.
- Encourage innovation to improve and diversify coronavirus vaccines.
- Continue to assess the durability afforded by different vaccine platforms.
- Promote coordination among immunologists, laboratory scientists, statisticians, clinicians, and computational biologists in efforts to conduct clinical trials for broadly protective coronavirus vaccines.
- Strategize as to how new technologies can be deployed on a global scale with greater equitable access.
- Continue to develop mechanisms to improve public communications regarding safety of coronavirus vaccines, such as tracking safety concerns from the public and developing consensus communication strategies to address them.
- Focus additional research on the effectiveness, side-effects, and durability of vaccines in special populations, such as children, pregnant women, the immunocompromised, and older people.
This section addresses the key barriers related to the availability of suitable animal models that are important to develop broadly protective coronavirus vaccines, and the need for these animal models to be standardized, validated, and well-characterized, and to mirror the human immune response and spectrum of clinical disease. The section also addresses the potential role of controlled human infection models to advance coronavirus vaccine R&D. Additional efforts that optimize the CHIM will broaden its utility, including identification of best practices and expanded use of standardized ethical and safety guidelines.
Animal Models
Issue: Multiple animal models will be needed to assess vaccines that protect against multiple coronaviruses.
Barriers
- SARS-CoV and SARS-CoV-2 bind to the hACE2 receptor; however, not all human coronaviruses bind to this site. MERS-CoV binds to DPP4, and the receptor site remains unknown for some of the viruses that cause milder disease in humans (Gralinski 2015). Therefore, several different animal models will likely be needed to study vaccines that protect against multiple coronaviruses of different genera or subgenera.
- Appropriate animal models for SARS-CoV and SARS-CoV-2 include Syrian hamsters, mice (e.g., transgenic mice, knock-in mice, mice transduced with adenovirus or adeno-associated virus expressing hACE2 or mice infected with mouse-adapted virus strains), and nonhuman primates (NHPs) (Muñoz-Fontela 2020, Qin 2022, Singh 2020, Casel 2021, Shou 2021, McCray 2007, Sun 2020, Wong 2022). While these various animal models can provide useful information, they all have important limitations (Qin 2022), including the following:
- Small-animal models offer several advantages, because they are readily available, can be handled with less effort and cost, and may be used in large numbers for stronger statistical power. The primary limitation is the intrinsic biological differences between humans and rodents or other small mammals.
- For NHPs, several issues limit their use, including: (1) coronavirus illness in NHPs is generally mild and does not recapitulate human pathology; (2) NHPs cost more (Gralinski 2015), which limits the number included in a study and lowers the statistical power; (3) most NHPs are outbred animals and vary widely in genetic backgrounds, which sometimes makes it difficult to interpret study outcomes (Trichel 2021); (4) ethical considerations constrain their use (Carvalho 2019); and (5) COVID-19 significantly increased demand for them, creating supply issues (Contreras 2021).
- Currently, virus stocks for different coronaviruses are limited. For example, 2d betacoronaviruses are not available for animal-model research, and stocks of 2c betacoronaviruses viruses other than MERS-CoV are very limited. (See Virology Applicable to Vaccine R&D.)
- Different animal models will be needed for studies with different aims (Wang 2021). For instance, when trying to determine whether SARS-CoV-2 still exists in the upper respiratory tract after vaccination or to study transmission, the Syrian hamster is a potential choice, although these animals develop limited clinical disease. For SARS-CoV-2–induced pulmonary disease, as well as a preliminary exploration of mucosal COVID-19 vaccines, hACE2 transgenic mice or the use of mouse-adapted viruses are potential options.
- The US FDA’s Animal Rule could be an appropriate regulatory pathway for facilitating approval of a broadly protective coronavirus vaccine through the use of animal studies if the requirements of other pathways cannot be met; however, achieving approval through the Animal Rule requires demonstrating efficacy in either multiple animal species or in a single well-characterized animal model (Brockhurst 2021, US FDA 2022).
- There is lack of standardization for experimentation and reporting for research involving NHPs (Witt 2021).
Gaps
- Research needs include the following:
- Standardized, validated, and well-characterized animal models (including NHPs and a panel of robust mouse models for human betacoronavirus disease) to evaluate and compare broadly protective coronavirus vaccines. Examples of parameters to consider include the challenge virus strain, dose, route, volume, and timing of challenge. Also, the appropriate clinical or virologic end points for each animal species need to be determined.
-
- Better characterization of coronavirus transmission dynamics in different animals to determine which animals are most appropriate for studying different SARS-CoV-2 variants or other coronaviruses.
- Animal models for SARS-CoV-2 VOCs to assess whether available vaccines offer protection against clinical disease (Fan 2022).
- Further elucidation of receptor sites for non-ACE2-binding coronaviruses (Dai 2020). (See Virology Applicable to Vaccine R&D.)
- Additional information—such as autopsy data from fatal human MERS-CoV infections—to determine which animal model best represents MERS-CoV in humans (Singh 2020).
- Animal models for studying bat-derived coronaviruses, such as group 2d betacoronaviruses.
- Animal models that can provide efficacy data against a wide breadth of viruses that represent a broad antigenic space. Examples include animal models that can “bracket” a broad range of immunity by showing that a vaccine can protect against infection with viruses that are least related—phylogenetically and antigenically—across a subgenus, multiple sub-genera, or different genera.
Issue: Animal models are needed that recapitulate the range of clinical features of coronavirus infection found in humans, including severe and lethal acute and chronic disease, and can address the impact of host factors on vaccine efficacy.
Barriers
- Most animal models exhibit limited lethality in response to coronavirus infection (Fan 2022, Kim 2022). This depends partially on the viral strain.
- Animal models are needed that are suitable for both antigenically naïve populations (i.e., infants and very young children) and antigenically experienced populations (i.e., adults and children who have been exposed to SARS-CoV-2 or vaccinated and those with exposures to coronaviruses causing mild illness), which complicates their development and application.
Gaps
- Research needs related to animal models include:
- Identification of animal models that recapitulate the severe and lethal forms of human SARS Co-V-2 infection (Muñoz-Fontela 2022).
- Identification of animal models that can assess disease for viruses that have not yet jumped the zoonotic barrier.
- Understanding potential differences in host-cell receptor pathways for viral binding.
- Further refinement of animal models to mimic different human conditions, such as route of infection, underlying morbidities, sex, advanced age, pregnancy, and immunocompromised status, that affect immune response to broadly protective coronavirus vaccines (Braxton 2021).
- Establishing animal models for SARS-CoV-2 that are suitable for antigenically experienced populations (i.e., those with prior coronavirus infection or vaccination).
- Experimentation in different animal models and using different emerging SARS-CoV-2 variants to ensure validity of research conclusions (Muñoz-Fontela 2022).
- Animal models (particularly mouse models) for assessing human T-cell responses (e.g., T helper cells [Th1 and Th2]) (Fan 2022, Jarnagin 2021).
- Animal models for assessing “long COVID” (Frere 2022), although numerous challenges exist for this, since long COVID has not been clearly defined and multiple pathologic pathways and chronic illnesses follow acute SARS-CoV-2 infection. This may be important in evaluating whether broadly protective coronavirus vaccines can lower the risk of long COVID/PASC.
Issue: Additional challenges exist to accurately assess broadly protective coronavirus vaccines in animal models.
Barriers
- Studying broadly protective coronavirus vaccines will require the availability of representative virus stocks for research in animal models, which may be challenging to obtain, particularly across different genera and subgenera of coronaviruses (Cohen J 2021).
- SARS-CoV, SARS-CoV-2, and MERS-CoV animal experimentation must be done in at least an animal BSL (ABSL)-3 laboratory, making work cumbersome and expensive. Furthermore, ABSL-3 laboratory space for NHP studies is limited (Hild 2021).
- A new SARS-CoV-2 variant might change the host range or affect the pathophysiology and response in certain animal models, such as Syrian hamsters. This in turn may render study in that animal difficult or lacking validity (Muñoz-Fontela 2022).
- Durable protection will be an important consideration for broadly protective coronavirus vaccines; however, duration of protection is difficult to study in animal models.
- As the COVID-19 pandemic continues and more of the human population is infected or vaccinated, the vast majority of humans are likely to have preexisting immunity to SARS-CoV-2, which may be difficult to mimic in animal models.
- As SARS-CoV-2 strains evolve and become more adapted to humans, they may become less able to infect animals or cause disease in animal models (McMahan 2022).
Gaps
- Head-to-head studies in animal models with multiple vaccine candidates could enhance understanding of vaccine-induced immunity.
- Ongoing efforts are needed to ensure that validated, reliable reagents; updated virus strains and stocks; and harmonized assays are available to the research community to improve understanding of the innate and adaptive immune responses against coronavirus infection in various animal models.
- Efforts are needed to adapt animal models to reflect preexisting immunity to SARS-CoV-2 in the human population (DeGrace 2022, Fan 2022).
- SARS-CoV-2 animal models are needed in which the virus replicates for extended periods to allow assessment for emergence of resistant variants against vaccines (Muñoz-Fontela 2022).
- Efforts are needed to ensure adequate, sustained supplies of animals and resources (including laboratory space) for research involving NHPs, particularly specific pathogen-free NHPs (Contreras 2021). Additionally, efforts are needed to conserve animal resources and develop strategies for good stewardship of such resources (Fan 2022).
Controlled Human Infection Model (CHIM)
Issue: The role of a CHIM in coronavirus vaccine research needs to be further clarified and defined.
Barriers
- The potential for severe disease or long-term sequelae (e.g., long COVID or PASC) following infection, although uncommon, may limit the utility of a CHIM studies to investigate SARS-CoV, SARS-CoV-2, and MERS-CoV (Williams 2022).
- The United Kingdom is the first, and remains the only, country to perform SARS-CoV-2 CHIM studies (Killingley 2022); therefore, recent experience with a CHIM for coronavirus research is limited. Efforts are under way to expand use of the CHIM to other countries.
- Similar to influenza virus and other pathogens, CHIM studies are limited to healthy adults without comorbidities and thus do not reflect potential outcomes in other populations (Sherman 2019).
- CHIM studies are currently limited to small sample sizes (Killingley 2022), which limits their utility to assess vaccine efficacy.
- Obtaining challenge viruses and the timelines needed to create challenge strains can be barriers to conducting CHIM research. GMP Delta and Omicron SARS-CoV-2 challenge viruses funded by the Wellcome Trust and the Bill & Melinda Gates Foundation will be made available to researchers with the capacity for CHIM studies and their rigorous safety requirements. An independent international Access Management Group as specified by the Wellcome Trust will provide oversight of these programs.
Gaps
- Additional research needs include the following:
- Clarification of the role of CHIM studies for evaluating broadly protective coronavirus vaccines, including their role in supporting vaccine licensure (Deming 2020, Giersing 2019, Sekhar 2020).
- Standardization of parameters for CHIM research in assessing broadly protective coronavirus vaccines.
- Development of best practices for using a CHIM in coronavirus vaccine research, including risk mitigation strategies that reflect a changing landscape of disease and therapies.
- Determining the potential impact of prior infection or vaccination against SARS-CoV-2 on CHIM studies of more broadly protective coronavirus vaccines and strategies to address this issue. It may be difficult or very resource intensive to find volunteers who are naïve to SARS-CoV-2 infection or vaccination.
- Regulatory harmonization for conducting CHIM studies.
- Capacity to run larger studies is needed so that efficacy trials can deliver timely results. This capacity gap includes BSL-3 quarantine facilities, expertise, and availability of effective monoclonal antibody therapies, antivirals, or other rescue therapeutics.
- Coronaviruses that cause mild disease in humans (human betacoronaviruses HKU1 and OC43 and human alphacoronaviruses 229E and NL63) may be suitable for use in a CHIM. Further clarification is needed regarding how such studies could contribute to coronavirus vaccine R&D (Morens 2022).
- Delta and Omicron programs are funded and under way to establish models in pre-immune volunteers, so data on the effect of prior immunity on infection by variants will be generated. For example, a dosing study using wild-type SARS-CoV-2 in both vaccinated and unvaccinated persons is under way (University of Oxford 2023).
- Studies in naïve participants are effectively no longer possible, as almost all adults have immunity from vaccination and/or infection.
- Improved international collaboration is needed so that products can be tested against different strains or viruses that may be available in various institutions around the world. Alignment of protocols and processes will allow meaningful comparison of results with different products and virus strains.
Strategic Goals and Aligned Milestones for Topic 4: Animal and Human Infection Models for Coronavirus Vaccine Research
Strategic Goal 4.1: Ensure that appropriate animal models are developed and available for conducting R&D for broadly protective coronavirus vaccines |
Milestone 4.1.a (High Priority): By 2024, develop a strategy to ensure that validated, reliable reagents; virus strains and stocks; and harmonized serologic assays are available for studying a broader range of coronaviruses in animal models, with an initial focus on additional sarbecoviruses (group 2b betacoronaviruses) and a wider variety of MERS-related merbecoviruses (group 2c betacoronaviruses). |
Milestone 4.1.b (High Priority): By 2024, convene an international workshop on animal models for studying broadly protective coronavirus vaccines. Examples of topics for the workshop include: (1) review existing animal models for SARS-CoV, SARS-CoV-2, MERS-CoV, and other coronaviruses; (2) determine which animal models are best suited for R&D of broadly protective coronavirus vaccines; (3) identify strategies to optimize the use of small-animal models (e.g., mice, hamsters, ferrets); (4) determine how best to optimize and reduce the use of NHPs for R&D efforts, particularly given their limited supply; (5) determine how to mimic preexisting immunity in animal models; (6) determine how animal models can be used to assess the impact of host genomics or the microbiome on vaccine performance, such as through the use of “dirty mice”; (7) determine the role of animal models in measuring mucosal immunity, breadth, and durability of vaccines; (8) determine the role of animal models in defining immune CoPs; (9) determine the role of animal models in studying long COVID/PASC; (10) identify gaps in the current animal model landscape; and (11) develop strategies and plans for meeting future animal-model research needs. |
Milestone 4.1.c: By 2025, identify the most suitable animal models for studying MERS-CoV in relation to coronavirus vaccine R&D. |
Milestone 4.1.d (High Priority): By 2025, ensure that standardized, validated, and well-characterized animal models are available to evaluate and compare broadly protective coronavirus vaccines. Examples of parameters to consider include the challenge virus strain; dose, route, volume, and timing of challenge; and animal responses to human-adapted variants. Immune history and prior exposure to ancestral coronaviruses should also be considered. The appropriate surrogate markers of clinical disease severity, such as weight loss or virus titers in the lungs, are needed for each animal species and for each virus sub-genus or relevant variant used to establish the model. |
Milestone 4.1.e: By 2025, conduct side-by-side comparisons of various animal models to determine transmission dynamics in different animals and which animals are most appropriate for studying different SARS-CoV-2 variants or other coronaviruses. |
Milestone 4.1.f: By 2026, conduct comparison studies of multiple vaccine candidates in different animal models, including small mammals and NHPs. |
Milestone 4.1.g: By 2026, conduct parallel studies of vaccine candidates in humans and NHPs that are aligned as closely as possible—such as by using similar dosing and schedules—to obtain information for immunobridging from animals to humans. |
Milestone 4.1.h: By 2027, ensure that standardized, validated, and well-characterized animal models are available that recapitulate the range of severe acute disease associated with human COVID-19, such as severe lung disease, coagulopathies, and neurologic disease (Muñoz-Fontela 2022). |
Strategic Goal 4.2: Establish the role of a CHIM in R&D for broadly protective coronavirus vaccines and optimize the model for vaccine research. |
Milestone 4.2.a: By 2023, conduct a workshop to clarify the role of CHIM studies for evaluating broadly protective coronavirus vaccines (Sekhar 2020). Examples of key issues include: (1) developing consensus on how CHIM models can be used for coronavirus vaccine research, (2) identifying strategies for studying the role of prior immunity from infection or vaccination on vaccine performance, (3) determining how CHIM studies can be used to assess mucosal immunity and mucosal inflammatory markers, (4) determining the role of the CHIM in establishing CoPs, (5) identifying strategies for assessing vaccine durability through CHIM studies, and (6) clarifying how CHIM studies involving coronaviruses that cause mild disease in humans could inform additional coronavirus vaccine R&D (Deming 2020, Morens 2022). |
Milestone 4.2.b: By 2024, determine the potential impact of prior infection or vaccination for SARS-CoV-2 on CHIM studies involving SARS-CoV-2 vaccines. |
Milestone 4.2.c (High Priority): By 2024, develop a set of best practices for using a CHIM in coronavirus vaccine research to include risk mitigation strategies that reflect the changing landscape of disease and therapies. |
Milestone 4.2.d (High Priority): By 2025, establish parameters, in coordination with global regulators, for using CHIM studies and immunobridging for licensure of candidate vaccines. |
Milestone 4.2.e (High Priority): By 2025—assuming candidate vaccines are available—standardize parameters for a CHIM model in assessing broadly protective coronavirus vaccines, such as determining appropriate strain selection (which may need to be defined contextually at the time), standardizing panels of immunologic assays and assay harmonization, identifying mucosal inflammatory markers, and harmonizing protocols as possible. |
Milestone 4.2.f: By 2026, establish international capacity and collaborative networks for conducting CHIM studies of broadly protective coronavirus vaccines. |
Milestone 4.2.g: By 2026—assuming candidate vaccines are available—determine the potential impact of prior infection or vaccination for SARS-CoV-2 on CHIM studies involving broadly protective coronavirus vaccines. |
Additional Research Priorities
- Further elucidate receptor sites for non-ACE2 binding coronaviruses to inform animal model development for coronavirus vaccine research (Dai 2020). (See Virology Applicable to Vaccine R&D for related milestones.)
- Conduct research to determine suitable animal models for bat-derived coronaviruses, such as group 2d betacoronaviruses.
- Further refine animal models over time to mimic different human conditions such as route of infection, underlying morbidities, sex, advanced age, and immunocompromised status that affect immune response to broadly protective coronavirus vaccines (Braxton 2021). Establishing animal models to study the possibility of VAED should also be considered.
- Determine on an ongoing basis the best strategies for using animal model studies in assessing the emergence of SARS-CoV-2 viral variants that can evade immune protection from vaccination or infection (Muñoz-Fontela 2022).
- Continue to explore how data from animal models or human infection models can be used to support vaccine licensure and what the parameters are for defining the role of such data. (See Vaccinology for related milestones.)
- Ensure, on an ongoing basis, adequate and sustained supplies of animals and resources—including laboratory space—for research involving NHPs, particularly specific pathogen-free NHPs (Contreras 2021).
- Employ single-cell transcriptomics in the CHIM to dissect cell-specific responses.
- Assess the role for animal models for studying long COVID/PASC in relation to vaccine R&D.
This section addresses the multiple policy and financing issues related to bringing broadly protective coronavirus vaccine candidates to market. Successful development and widespread availability of new vaccines will require reinvigorating and sustaining a high level of political commitment and investment in vaccine R&D, as well as strengthening global manufacturing and distribution to ensure equitable access. Detailed analyses that capture the wide range of potential social and economic benefits of broadly protective coronavirus vaccines will inform policymaking and essential global investment.
Issue: Multiple market forces work against bringing broadly protective coronavirus vaccines to the global community in high-, middle-, and low-income countries.
Barriers
- The current coronavirus vaccine R&D field is filled with an array of patents, contracts and agreements; social and economic inequities; uneven geographic distribution of manufacturing capacity; and unstable funding.
- R&D of new vaccines is exceedingly expensive. Recently, governments and foundations provided billions of dollars to bring SARS-CoV-2 vaccines to market; however, the political will and public interest for continued funding are challenging to maintain in a landscape where there is always the next variant, virus, or pandemic threat (Lancet Commission 2021), and public support for such large-scale investments is diminishing (Branswell 2022). Also, once a crisis has passed, government funding will be more difficult to obtain, since governments face pressures to address urgent crises rather than long-term strategies.
- Vaccines can be a winner-takes-all (or most) market, with a significant advantage to being first to market. This is especially true in pandemic or epidemic situations, where the first vaccine to demonstrate efficacy is fully purchased by governments before other candidate vaccines have had a chance to complete their clinical development. The rapidly waning immunity of early SARS-CoV-2 vaccines and decreased vaccine efficacy against variants, however, may reduce some of the first-to-market advantage for current coronavirus vaccines.
- Unless problems are noted with a vaccine, there is little incentive to invest in better or next-generation vaccines (Agarwal 2022). With SARS-CoV-2 vaccines, the financial risks and benefits of the current situation tend to favor minor changes to existing technologies rather than investment in novel technologies. For example, one current commercial model is to create boosters specific to new variants as they arise, using existing platforms. This model may play into the status quo of creating strain-specific vaccines, rather than expanding vaccine R&D to generate broadly protective vaccines.
- Unless costs are absorbed by governments or other funding bodies, companies face a high opportunity cost when it comes to focusing on vaccines rather than other pharmaceuticals with a likely higher per-unit profit, ongoing use, and stable demand (Cernuschi 2022).
- Coronavirus vaccines face a large global market that is dominated by a few large purchasers. A large market can be seen as both an opportunity and a hindrance for private investment into new vaccines. For example, a large market in high-income countries (HICs) can lead to higher revenue, but a larger market can also result in lower per-unit vaccine costs as governments or other large global buyers, such as Gavi, the Vaccine Alliance, negotiate extensive contracts, which can be market-shaping and lead to less flexibility in pricing (Haugen 2021, Agarwal 2022, Monrad 2021).
- Ensuring global equity in vaccine access will need to address the current geographic concentration of vaccine R&D, manufacturing, and purchasing power of HICs, which can lead to gross inequities in the vaccine market. Organizations such as Gavi played a critical role in securing COVID-19 vaccines for the world’s poorest countries through the newly established COVID-19 Vaccines Global Access (COVAX) Facility. It remains unclear what the role of Gavi and the COVAX Facility will be for the procurement of future broadly protective coronavirus vaccines for LMICs.
- Some middle-income countries (MICs), which may not be eligible for assistance from organizations such Gavi, may lack access to vaccines that lower- and higher-income countries are able to obtain (Cernuschi 2022).
- Maximizing the potential benefit of vaccination relies on global demand and vaccine uptake. This will largely be determined by whether such vaccines will be used prophylactically on a routine basis (thereby creating a large demand) or whether they will be stockpiled for outbreak response (leading to a relatively uncertain demand). Vaccine developers remember times when the vaccine markets lacked demand, making recouping of development costs less certain (Kumraj 2022).
Gaps
- A multitude of ongoing efforts in basic and applied research, laboratory systems, research infrastructure, and global capacity-building are working to bring broadly protective coronavirus vaccines to a global market. However, efforts are not coordinated, broadly shared, or designed for efficiency and avoidance of duplication.
- Unlike the beginning of the COVID-19 pandemic, when there was considerable urgency to invest in novel products, at this stage, a focused set of incentives may be needed to encourage novel vaccine technologies that may be superior to first-generation vaccines. However, until novel technologies are shown to be superior to first-generation vaccines, vaccines that have a proven track-record cannot be abandoned or ignored.
- Alongside the “push” incentives of government funding and non-monetary drivers, “pull” incentives, such as advanced market commitments that signal a predictable and sufficient market, are needed in HICs and LMICs to drive products toward approval, manufacturing, and availability. Strategies to support these incentives are needed.
Issue: Intellectual property rights are a critical aspect of vaccine innovation, yet come at a cost.
Barriers
- New vaccine candidates will likely be based on a series of patented technologies, many of which already exist. Many different patents apply to vaccines from development to components to manufacturing to delivery (WIPO 2022).
- Although governments heavily fund academic and corporate R&D, the resulting intellectual property rights of these public funds end up in private sector, non-governmental domains. The resulting intellectual property rights are an important incentive for commercial investment in vaccine development, but the public sector may be reluctant to increase public investment when it is unclear if there will be a commensurate public access to intellectual property established through the use of public monies (Rees 2022b).
- Only a partial picture is available of patents that exist surrounding next-generation coronavirus vaccines.
- Four hundred and seventeen patents related to COVID-19 vaccine development were filed from 2020 through September 30, 2021 (WIPO 2022). Patent publications, however, can take 18 months to be published (Alshrari 2022, Kitsara 2022, WIPO 2022). The time to patent publication varies by country, from 7.7 months in China to 18.8 in the United States and 18.9 in Japan (WIPO 2022). Because of the lag in entering the public domain, these 417 are only an early indication of the patent activity surrounding COVID-19 vaccines, as well as technology related to vaccine research, development, testing, and production.
- Negotiating licensing agreements and understanding the intellectual property landscape can be quite costly and require expertise, which may cause vaccine developers to hesitate in pursuing a new approach.
Gaps
- The future application of World Trade Organization (WTO) agreements, including Trade Related Aspects of Intellectual Property Rights (TRIPS) flexibilities for public health emergencies, is uncertain and needs clarification.
- Awards of public monies for R&D do not always include clauses to improve intellectual property access for smaller developers, those in LMICs, and more broadly during times of public health need. Similarly, with few exceptions (e.g., CEPI), public investment does not compel developers to commit to ensuring access in LMICs if their product is successfully developed.
- The long-term outcomes of voluntary licensing and sharing being undertaken during the COVID-19 pandemic are unknown. It is unclear what will happen to intellectual property rights not currently being enforced when the pandemic is “over.”
- Efforts are needed to clarify the role of patent pools, such as the WHO’s COVID-19 Technology Access Pool (C-TAP), and vaccines capitalizing on established technologies that are not patent-protected (Hotez 2020, WHO 2020a).
Issue: Timely access to broadly protective coronavirus vaccines will require greater manufacturing capacity.
Barriers
- If a new coronavirus emerges to cause another pandemic, rapid access globally to either a strain-specific vaccine or to broadly protective coronavirus vaccines will be critical for mitigating pandemic impact. A global concentration of manufacturing and regulatory capacity exists in HICs and in some populous countries, guaranteeing them a large market. Furthermore, the current vaccine industry can, during a public health emergency with vaccine shortages, become protectionist either because of government constraints on vaccine exports or because of tiered pricing structures that favor HICs. In the case of COVID-19, both considerations likely contributed to the inequity in vaccine access (Cernuschi 2022).
- Successful technology transfer is complex and requires trusted partners with expertise and capacity, long-term human and financial investment, and political will (Kumraj 2022).
- Countries have highly variable levels of regulatory capacity to monitor GCP, GMP, and GLP, and very few regulatory authorities in LMICs have received a WHO maturity level 3 for vaccines, which is required if locally manufactured vaccines are to be considered for the global market (Kumraj 2022).
- Intellectual property waivers alone may not be as successful as good and complete technology transfers based on manufacturing capacity and expertise (Prasad 2022).
- Patent holders are looking for trusted partners for technology transfer to ensure there is the expertise and experience to produce high-quality, safe vaccines that can pass regulatory approval (Kahla 2022, Nohynek 2022, Rizvi 2022).
- Manufacturing capacity is not merely an issue of building the facilities and expertise, but also the ability to maintain this capacity and remain financially sustainable over time, particularly during non-pandemic times.
Gaps
- It is unknown how voluntary technology transfers, pledges to not enforce patents, WTO actions, TRIPS flexibilities, and licensing agreements will play out in the next phases of the COVID-19 pandemic or as the pandemic wanes.
- Funding at-risk manufacturing, or scaling up dose production ahead of clinical trial completion or vaccine regulatory approval, may be a way to speed up the availability of new vaccines in the event of a public health emergency (Sampat 2021). However, there is currently little appetite for these mechanisms, and how this could be used to promote broadly protective coronavirus vaccines is unknown; thus, further exploration of mechanisms to address these issues is warranted.
- WHO and its partners have established the mRNA Technology Transfer Hub, the global biomanufacturing training hub in the Republic of Korea, and the Global Benchmarking Tool for regulatory authorities to address global manufacturing capacity (WHO 2021, WHO 2022c, WHO 2022d). However, efforts are still needed to operationalize these programs, generate sustainable business models, expand engagement of companies with the most advanced capacities, and expand efforts to other vaccine platforms beyond mRNA technologies (Gavi 2022).
Strategic Goals and Aligned Milestones for Topic 5: Policy and Financing
Strategic Goal 5.1. Establish and convey the value of sustained financial support and demand for developing broadly protective coronavirus vaccines. |
Milestone 5.1.a (High Priority): By 2024, develop and disseminate a detailed economic case for broadly protective coronavirus vaccines through a full value of vaccine assessment (FVVA) or a series of detailed cost-benefit analyses for vaccines from SARS-CoV-2 variant-proof vaccines to more broadly protective coronavirus vaccines. These assessments will need to include a multitude of perspectives (e.g., health payers, economic, and societal) at a number of levels (e.g., global, national, and regional) and take into account varying contexts (e.g., demographics, healthcare capacity, competing health priorities) and the potential pathways for deployment, such as routine, preventive use or reactive outbreak control (Giersing 2021). |
Milestone 5.1.b: By 2024, develop targeted communications and advocacy strategies and necessary communication tools that build on the FVVA or cost-effectiveness analyses and provide information on the economic costs, risks of future coronavirus threats, and the need for continuing investment in coronavirus vaccine R&D. |
Milestone 5.1.c (High Priority): By 2024, convene a meeting of vaccine investors, purchasers (including governments and large global institutions), producers, and governmental representatives aimed at exploring strategies for providing a reliable marketplace and financial model for broadly protective coronavirus vaccines. Meeting participants will assess the current push (e.g., grants, subsidies) and pull incentives (e.g., advance market commitments) and appropriate thresholds to move from push to pull, as well as establish a pricing model in line with the PPCs that can be anticipated for vaccines of various characteristics, such as the number of doses required, stability, duration of protection, and level of protection. |
Milestone 5.1.d: By 2025, explore strategies for developing a coordinated approach to generate sustainable, long-term funding, involving public and private partners, aimed at supporting R&D for broadly protective coronavirus vaccines. |
Strategic Goal 5.2. Reassess the current landscape of intellectual property rights to improve information sharing involving new technologies. |
Milestone 5.2.a: By 2023, develop a consensus vision for how licensing of intellectual property rights derived from academic or publicly funded research can address inequity and adopt global equitable access clauses from the earliest stage of research. |
Milestone 5.2.b: By 2024, initiate a resource center to support licensing negotiations and intellectual property capacity building by scientists who may not have the resources or background knowledge to effectively achieve access to patented technologies. |
Strategic Goal 5.3. Build a sustainable and more balanced geographic distribution of manufacturing capacity with expertise to manufacture high-quality vaccines for local use. |
Milestone 5.3.a: By 2024, establish a consensus on what is acceptable geographic distribution for vaccine manufacturing and potential pathways for manufacturing to transition from variant-specific SARS-CoV-2 vaccines to broadly protective coronavirus vaccines. |
Milestone 5.3.b: By 2027, provide the necessary resources to ensure that 100% of countries with vaccine manufacturing capacity are able to at least partially implement the WHO inspection indicators, as defined by the WHO Global Benchmarking Tool (WHO 2021). |
Milestone 5.3.c: By 2028, through global initiatives supporting the manufacture of various vaccine-platform technologies: (1) design and build manufacturing sites meeting the GMP criteria for vaccines, (2) transfer expertise for various vaccine platforms and other relevant technologies, and (3) begin producing vaccines in at least several new locations, with a plan to maintain capacity over time and through inter-pandemic years (Gavi 2022, Medicines Patent Pool 2022). |
Additional Research Priorities
- Coordinate efforts to address the various challenges facing financing of R&D for broadly protective coronavirus vaccines.
- Maintain increased global sharing and communication across scientists, vaccine developers, manufacturers, funders, and government bodies.
- Review the experience with the TRIPS agreements and voluntary non-enforcement of intellectual property rights during the early years of the COVID-19 pandemic.
- Continue to gather data on the role and impact of coronavirus vaccines (including vaccine effectiveness) to build vaccine demand, which will in turn affect policy development.
Adams LE, Leist SR, Dinnon KH, et al., Fc mediated pan-sarbecorvirus protection after alphabirus vector vaccination. (Preprint) bioRxiv 2022 Nov 22;11.28.518175 [Full text]
Agarwal R, Gaule P. What drives innovation? Lessons from COVID-19 R&D. J Health Econ 2022 Mar;82:102591 [Full text]
Aguilar-Bretones M, Fouchier RA, Koopmans MP, et al. Impact of antigenic evolution and original antigenic sin on SARS-CoV-2 immunity. J Clin Invest 2023 Jan 3;133(1):e162192 [Full text]
Ahmed-Hassan H, Sisson B, Shukla RK, et al. Innate immune responses to highly pathogenic coronaviruses and other significant respiratory viral infections. Front Immunol 2020 Aug 18;11:1979 [Full text]
Alkhovsky S, Lenshin S, Romashin A, et al. SARS-like coronaviruses in horseshoe bats (Rhinolophus spp) in Russia, 2020. Viruses 2022 Jan 9;14(1):113 [Full text]
Alshrari AS, Hudu SA, Imran M, et al. Innovations and development of Covid-19 vaccines: A patent review. J Infect Public Health 2022 Jan;15(1):123-31 [Full text]
Altmann DM, Boyton RJ. COVID-19 vaccination: the road ahead. Science 2022 Mar 10;375(6585):1127-32 [Full text]
Ansariniya H, Seifati SM, Zaker E, et al. Comparison of immune response between SARS, MERS, and COVID-19 infection, perspective on vaccine design and development. Biomed Res Int 2021 Jan 22:8870425 [Full text]
Anthony SJ, Johnson CK, Greig DJ, et al. Global patterns in coronavirus diversity. Virus Evol 2017 Jan;3(1):vex012 [Full text]
Arevalo CP, Bolton MJ, Le Sage V, et al. A multivalent nucleoside-modified mRNA vaccine against all known influenza virus subtypes. Science 2022 Nov 25;378(6622):899-904 [Abstract]
Azzi L, Dalla Gasperina D, Veronesi G, et al. Mucosal immune response in BNT162b2 COVID-19 vaccine recipients. eBioMedicine 2022 Jan;75:103788 [Full text]
Babady NE, Burckhardt RM, Krammer F, et al. Building a resilient scientific network for COVID-19 and beyond. mBio 2022 Sep-Oct;13(5):e02223-22 [Full text]
Baric R. Major challenges with development of pan-coronavirus vaccines. Presentation at WHO consultation on COVID-19 vaccines research—advancing the development of pan-sarbecovirus vaccines. 2022 Mar 25 [Presentation at 13:54:00]
Bean DJ, Sagar M. Family matters for coronavirus disease and vaccines. (Commentary) J Clin Invest 2021 Dec 15;131(24):e155615 [Full text]
Beasley DWC, Brasel TL, Comer JE. First vaccine approval under the FDA Animal Rule. NPJ Vaccines 2016;1:16013 [Full text]
Begum J, Mir NA, Dev K, et al. Challenges and prospects of COVID-19 vaccine development based on the progress made in SARS and MERS vaccine development. Transbound Emerg Dis 2021 May;68(3):1111-24 [Full text]
Belyakov IM, Ahlers JD. What role does the route of immunization play in the generation of protective immunity against mucosal pathogens? J Immunol 2009 Dec 1;183(11):6883-92 [Full text]
Bhattacharya, D, Instructing durable humoral immunity for COVID-19 and other vaccinable diseases. Immunity 2022 Jun 14;55(6):945-64 [Full text]
Bigay J, Le Grand R, Martinon F, et al. Vaccine-associated enhanced disease in humans and animal models: lessons and challenges for vaccine development. Front Microbiol 2022 Aug 10;13:932408 [Full text]
Bolles M, Deming D, Long K, et al. A double-inactivated severe acute respiratory syndrome coronavirus vaccine provides incomplete protection in mice and induces increased eosinophilic proinflammatory pulmonary response upon challenge. J Virol 2011 Dec;85(23):12201-15 [Full text]
Bots SH, Riera-Arnau J, Belitser SV, et al. Myocarditis and pericarditis associated with SARS-CoV-2 vaccines: a population-based descriptive cohort and a nested self-controlled risk interval study using electronic health care data from four European countries. Front Pharmacol 2022 Nov 24;13:1038043 [Full text]
Branswell H. The COVID-19 vaccine market is getting crowded—as demand begins to wane. Stat 2022 Mar 21 [Full text]
Braxton AM, Creisher PS, Ruiz-Bedoya CA, et al. Hamsters as a model of severe acute respiratory syndrome coronavirus-2. Comp Med 2021 Oct;71(5):398-410 [Full text]
Britto C, Alter G. The next frontier in vaccine design: blending immune correlates of protection into rational vaccine design. Curr Opin Immunol 2022 Aug 13;78:102234 [Full text]
Brockhurst JK, Villano JS. The role of animal research in pandemic responses. Comp Med 2021 Oct;71(5):359-68 [Full text]
Brüssow H. COVID-19: vaccination problems. Environ Microbiol 2021 Jun;23(6):2878-90 [Full text]
Cameroni E, Bowen JE, Rosen LE, et al. Broadly neutralizing antibodies overcome SARS-CoV-2 Omicron antigenic shift. Nature 2022 Feb 24;602(7898):664-70 [Full text]
Carmen JM, Shrivastava S, Lu Z, et al. SARS-CoV-2 ferritin nanoparticle vaccine induces robust innate immune activity driving polyfunctional spike-specific T cell responses. NPJ Vaccines 2021 Dec 13;6:151 [Full text]
Carvalho C, Gaspar A, Knight A, et al. Ethical and scientific pitfalls concerning laboratory research with non-human primates, and possible solutions. Animals (Basel) 2019 Jan;9(1):12 [Full text]
Casel MAB, Rollon RG, Choi YK. Experimental animal models of coronavirus infections: strengths and limitations. Immune Netw 2021 Apr 26;21(2):e12 [Full text]
Cavaleri M. What are the critical preclinical challenges and how could they be addressed for future candidates before the next pandemic? Panel discussion at WHO: Scientific strategies from recent outbreaks to help us prepare for pathogen X. Day 2, session 5. 2022 Aug 30 [Presentation at 2:38:00]
CBD (Convention on Biological Diversity). The Nagoya protocol on access to genetic resources and the fair and equitable sharing of benefits arising from their utilization. Secretariat of the Convention on Biological Diversity, United Nations Environmental Programme. 2011 [Web page with links to full text]
Cernuschi T, Malvolti S, Hall S, et al. The quest for more effective vaccine markets—opportunities, challenges, and what has changed with the SARS-CoV-2 pandemic. Vaccine. 2022 Oct 21 [Full text]
Cervantes M, Hess T, Morbioli GG, et al. The ACE-2 receptor accelerates but is not biochemically required for SARS-CoV-2 membrane fusion. (Preprint) bioRxiv 2022 Oct 24:513347 [Full text]
Chen L, Liu B, Yang J, et al. DBatVir: the database of bat-associated viruses. Database (Oxford) 2014 Mar18;2014:bau021 [Full text]
Chen Z, Azman AS, Chen X, et al. Global landscape of SARS-CoV-2 genomic surveillance and data sharing. Nat Genet 2022 Apr;54(4):499-507 [Full text]
Chiu NC, Chi H, Tu YK, et al. To mix or not to mix? A rapid systematic review of heterologous prime–boost covid-19 vaccination. Expert Rev Vaccines 2021 Oct;20(10):1211-20 [Full text]
CIDRAP. Influenza vaccines R&D roadmap. 2021 Sep [Full text]
Cohen AA, Gnanapragasam PNP, Lee YE, et al. Mosaic nanoparticles elicit cross-reactive immune responses to zoonotic coronaviruses in mice. Science 2021 Feb 12;371(6530):735-41 [Full text]
Cohen AA, van Doremalen N, Greaney AJ, et al. Mosaic RBD nanoparticles protect against multiple sarbecovirus challenges in animal models. (Preprint) bioRxiv 2022 Mar 28 [Full text]
Cohen J. The dream vaccine: why stop at just SARS-CoV-2? Vaccines in development aim to protect against many coronaviruses at once. Science 2021 Apr 16;372(6539):227-31 [Full text]
Collier AY, Brown CM, McMahan KA, et al. Characterization of immune responses in fully vaccinated individuals after breakthrough infection with the SARS-CoV-2 delta variant. Sci Transl Med 2022 Apr 20;14(641):eabn6150 [Full text]
Contreras MA, Arnegard, ME, Chang MC, et al. Nonhuman primate models for SARS-CoV-2 research: managing demand for specific-pathogen-free (SPF) animals. (Comment) Lab Anim 2021 Aug;50:200-1 [Full text]
Cotten M, Robertson DL, Phan MVT. Unique protein features of SARS-CoV-2 relative to other sarbecoviruses. Virus Evol 2021;7(2):veab067 [Full text]
Crowe JE Jr. Human antibodies for viral infections. Annu Rev Immunol 2022 Apr 26;40:349-86 [Abstract]
Cucinotta D, Vanelli M. WHO declares COVID-19 a pandemic. Acta Biomed 2020;91(1):157-60 [Full text]
Cunningham AA, Daszak P, Wood JLN. One Health, emerging infectious diseases and wildlife: two decades of progress? Philos Trans R Soc Lond B Biol Sci 2017;372(1725):20160167 [Full text]
Dai L, Zheng T, Xu K, et al. A universal design of betacoronavirus vaccines against COVID-19, MERS, and SARS. Cell 2020 Aug 6;182(3):722-33 [Full text]
Dangi T, Palacio N, Sanchez S, et al. Cross-protective immunity following coronavirus vaccination and coronavirus infection. J Clin Invest 2021 Dec 15;131(24):e151969 [Full text]
DeGrace MM, Ghedin E, Frieman MB, et al. Defining the risk of SARS-CoV-2 variants on immune protection. (Perspective) Nature 2022;605:640-52 [Full text]
Deming ME, Michael NL, Robb M, et al. Accelerating development of SARS-CoV-2 vaccines—the role for controlled human infection models. (Perspective) N Engl J Med 2020 Sep 3;383(10):e63 [Full text]
Diamond MS, Kanneganti TD. Innate immunity: the first line of defense against SARS-CoV-2. Nat Immunol 2022 Feb;23(2):165-176 [Full text]
DiPiazza AT, Leist SR, Abiona OM, et al. COVID-19 vaccine mRNA-1273 elicits a protective immune profile in mice that is not associated with vaccine-enhanced disease upon SARS-CoV-2 challenge. Immunity 2021 Aug 10;54(8):1869-82.e6 [Full text]
ECDC (European Centre for Disease Prevention and Control). MERS-CoV worldwide overview: Situation update. 5 Dec 2022. Accessed 2023 Jan 9 [Web page]
Economist. All around the world, covid surveillance is faltering. Economist 2023 Jan 7 [Introduction]
Eguia RT, Crawford KHD, Stevens-Ayers T, et al. A human coronavirus evolves antigenically to escape antibody immunity. PLOS Pathog 2021 Apr 8;17(4):e1009453 [Full text]
El-Sayed A, Kamel M. Coronaviruses in humans and animals: the role of bats in viral evolution. Environ Sci Pollut Res Int 2021;28(16):19589-600 [Full text]
Espinoza C, Alarcón M. The immune response to SARS-CoV-2: mechanisms, aging, sequelae, and vaccines. Mini Rev Med Chem 2022;22(16):2166-85 [Full text]
Fan C, Wu Y, Rui X, et al. Animal models for COVID-19: advances, gaps and perspectives. Signal Transduct Target Ther 2022 Jul 7;7(1):220 [Full text]
Farley J. Can major regulatory principles be laid out in advance? Panel discussion at WHO: Scientific strategies from recent outbreaks to help us prepare for pathogen X. Day 2, session 4. 2002 Aug 30 [Presentation at 2:35:00]
Forni D, Cagliani R, Clerici M, et al. Molecular evolution of human coronavirus genomes. Trends Microbiol 2017 Jan;25(1):35-48 [Full text]
Frere JJ, Serafini RA, Pryce KD, et al. SARS-CoV-2 infection in hamsters and humans results in lasting and unique systemic perturbations after recovery. Sci Transl Med 2022 Sep 28;14(664):eabq3059 [Full text]
Frutos R, Serra-Cobo J, Pinault L, et al. Emergence of bat-related betacoronaviruses: hazard and risks. Front Microbiol 2021 Mar 15;12:591535 [Full text]
Gaebler C, Wang Z, Lorenzi JCC, et al. Evolution of antibody immunity to SARS-CoV-2. Nature 2021 Mar;591(7851):639-44 [Full text]
Gartlan C, Tipton T, Salguero FJ, et al. Vaccine-associated enhanced disease and pathogenic human coronaviruses. Front Immunol 2022 Apr 4;13:882972 [Full text]
Gavi, the Vaccine Alliance. Expanding sustainable vaccine manufacturing in Africa: priorities for support. 2022 Nov [Full text]
Ghai RR, Carpenter A, Liew AY, et al. Animal reservoirs and hosts for emerging alphacoronaviruses and betacoronaviruses. Emerg Infect Dis 2021;27(4):1015-22 [Full text]
Giersing B, Shah N, Kristensen D, et al. Strategies for vaccine-product innovation: creating an enabling environment for product development to uptake in low- and middle-income countries. Vaccine 2021 Dec 3;39(49):7208-19 [Full text]
Giersing BK, Porter CK, Kotloff K, Neels P, et al. How can controlled human infection models accelerate clinical development and policy pathways for vaccines against Shigella? Vaccine 2019 Aug 7;37(34):4778-83 [Full text]
Gilbert PB, Donis RO, Koup RA, et al. A Covid-19 milestone attained—a correlate of protection for vaccines. N Engl J Med 2022 Dec 15;387(24):2203-6 [Full text] (Gilbert 2022a)
Gilbert PB, Montefiori DC, McDermott AB, et al. Immune correlates analysis of the mRNA-1273 COVID-19 vaccine efficacy clinical trial. Science 2022 Jan 7;375(6576):43-50 [Full text] (Gilbert 2022b)
GISAID (Global Initiative on Sharing All Influenza Data). Accessed 2022 Oct 10 [Web page]
Goldblatt D, Alter G, Crotty S, et al. Correlates of protection against SARS-CoV-2 infection and COVID-19 disease. Immunol Rev 2022 Sep;310(1):6-26 [Full text] (Goldblatt 2022a)
Goldblatt D, Fiore-Gartland A, Johnson M, et al. Towards a population-based threshold of protection for COVID-19 vaccines. Vaccine 2022 Jan 21;40(2):306-15 [Full text] (Goldblatt 2022b)
Goraichuk IV, Arefiev V, Stegniy BT, et al. Zoonotic and reverse zoonotic transmissibility of SARS-CoV-2. Virus Res 2021;302:198473 [Full text]
Gralinski LE, Baric RS. Molecular pathology of emerging coronavirus infections. J Pathol 2015;235(2):185-95 [Full text]
Grifoni A, Sette A. From alpha to omicron: the response of T cells. Curr Res Immunol 2022;3:146-50 [Full text]
Hachmann NP, Miller J, Collier AY, et al. Neutralization escape by SARS-CoV-2 Omicron subvariants BA.2.12.1, BA.4, and BA.5. (Correspondence) N Engl J Med 2022 Jul 7;387(1):86-8 [Full text]
Halfmann PJ, Frey SJ, Loeffler K, et al. Multivalent S2-based vaccines provide broad protection against SARS-CoV-2 variants of concern and pangolin coronaviruses. eBioMedicine 2022 Dec;86:104341 [Full text]
Harrison PW, Lopez R, Rahman N, et al. The COVID-19 Data Portal: accelerating SARS-CoV-2 and COVID-19 research through rapid open access data sharing. Nucleic Acids Res 2021 Jul 2;49(W1):W619-23 [Full text]
Harvey WT, Carabelli AM, Jackson B, et al. SARS-CoV-2 variants, spike mutations and immune escape. Nat Rev Microbiol 2021;19(7):409-24 [Full text]
Haugen HM. Does TRIPS (Agreement on Trade-Related Aspects of Intellectual Property Rights) prevent COVID-19 vaccines as a global public good? J World Intellect Prop 2021 Jul;24(3-4):195-220 [Full text]
Hauser BM, Sangesland M, St Denis KJ, et al. Rationally designed immunogens enable immune focusing following SARS-CoV-2 spike imprinting. Cell Rep 2022 Mar 22;38(12):110561 [Full text]
Hild SA, Chang MC, Murphy SJ, et al. Nonhuman primate models for SARS-CoV-2 research: infrastructure needs for pandemic preparedness. (Comment) Lab Anim 2021 Jun;50(6):140-1 [Full text]
Hodgson SH, Mansatta K, Mallett G, et al. What defines an efficacious COVID-19 vaccine? A review of the challenges assessing the clinical efficacy of vaccines against SARS-CoV-2. Lancet Infect Dis 2021 Feb;21(2):e26-e35 [Full text]
Holmes EC, Goldstein SA, Rasmussen AL, et al. The origins of SARS-CoV-2: a critical review. Cell 2021 Sep 16;184(19):4848-56 [Full text]
Hotez PJ, Bottazzi ME. Developing a low-cost and accessible COVID-19 vaccine for global health. PLOS Negl Trop Dis 2020 Jul 29;14(7):e0008548 [Full text]
Houtman J, Shultz L, Rivera JM, et al. Variants, sublineages, and recombinants: the constantly changing genome of SARS-CoV-2. The Rockefeller Foundation. 2022 Mar 25 [Full text]
Hsieh CL, Werner AP, Leist SR, et al. Stabilized coronavirus spike stem elicits a broadly protective antibody. Cell Rep 2021 Nov 2;37(5):109929 [Full text]
Huang AT, Garcia-Carreras B, Hitchings MDT, et al. A systematic review of antibody mediated immunity to coronaviruses: kinetics, correlates of protection, and association with severity. Nat Commun 2020 Sep 17;11:4704 [Full text]
Hu B, Guo H, Zhou P, et al. Characteristics of SARS-CoV-2 and COVID-19. Nat Rev Microbiol 2021 Mar;19(3):141-54 [published correction: Nat Rev Microbiol 2022 May;20(5):315] [Full text]
Hu B, Zeng LP, Yang XL, et al. Discovery of a rich gene pool of bat SARS-related coronaviruses provides new insights into the origin of SARS coronavirus. PLOS Pathog 2017 Nov 30;13(11):e1006698 [Full text]
Inoue T, Shinnakasu R, Kurosaki T. Generation of high quality memory B cells. Front Immunol 2022 Jan 12;12:825813 [Full text]
INSDC (International Nucleotide Sequence Database Collaboration). Accessed 2023 Jan 12 [Website]
Irving AT, Ahn M, Goh G, et al. Lessons from the host defenses of bats, a unique viral reservoir. Nature 2021 Jan;589(7842):363-70 [Full text]
Iwasaki A. Exploiting mucosal immunity for antiviral vaccines. Annu Rev Immunol 2016 May 20;34:575-608 [Abstract]
Jang YH, Seong BL. Call for a paradigm shift in the design of universal influenza vaccines by harnessing multiple correlates of protection. Expert Opin Drug Discov 2020 Dec;15(12):1441-55 [Abstract]
Jarnagin K, Alvarez O, Shresta S, et al. Animal models for SARS-Cov2/Covid19 research. (Commentary) Biochem Pharmacol 2021 Jun;188:114543 [Full text]
Johns Hopkins University & Medicine. Coronavirus Resource Center. Mortality in the most affected countries. Accessed 2022 Dec 28 [Website]
Joyce MG, King HAD, Elakhal-Naouar I, et al. A SARS-CoV-2 ferritin nanoparticle vaccine elicits protective immune responses in nonhuman primates. Sci Transl Med 2022 Feb 16;14(632):eabi5735 [Full text]
Kahla K. Access and accountability. Presentation at WHO: COVID-19 research and innovation forum: powering the world’s pandemic response—now and in the future. Day 2, Pt 1. 2022 Feb 24-25 [Presentation at 2:20:00]
Kaplonek P, Cizmeci D, Fischinger S, et al. mRNA-1273 and BNT162b2 COVID-19 vaccines elicit antibodies with differences in Fc-mediated effector functions. Sci Transl Med 2022 Mar 29:eabm2311 [Full text]
Karczmarzyk K, Kesik-Brodacka M. Attacking the intruder at the gate: prospects of mucosal anti SARS-CoV-2 vaccines. Pathogens 2022 Jan 19;11(2):117 [Full text]
Karim SSA. Vaccines and SARS-CoV-2 variants: the urgent need for a correlate of protection. (Correspondence) Lancet 2021 Apr 3;397(10281):1263-4 [Full text]
Kent SJ, Khoury DS, Reynaldi A, et al. Disentangling the relative importance of T cell responses in COVID-19: leading actors or supporting cast? Nat Rev Immunol 2022 Jun;22(6):387-97 [Full text]
Keusch GT, Amuasi JH, Anderson DE, et al. Pandemic origins and a One Health approach to preparedness and prevention: solutions based on SARS-CoV-2 and other RNA viruses. Proc Natl Acad Sci USA 2022 Oct 18;119(42):e2202871119 [Full text]
Khoury DS, Cromer D, Reynaldi A, et al. Neutralizing antibody levels are highly predictive of immune protection from symptomatic SARS-CoV-2 infection. Nat Med 2021 Jul;27(7):1205-11 [Full text]
Khoury DS, Schlub TE, Cromer D, et al. Correlates of protection, thresholds of protection, and immunobridging in SARS-CoV-2 infection. (Preprint) medRxiv 2022 Jun 6 [Full text]
Killingley B, Mann AJ, Kalinova M, et al. Safety, tolerability and viral kinetics during SARS-CoV-2 human challenge in young adults. Nat Med 2022;28(5):1031-41 [Full text]
Kim YI, Casel MAB, Choi YK. Transmissibility and pathogenicity of SARS-CoV-2 variants in animal models. J Microbiol 2022 Mar;60(3):255-67 [Full text]
Kistler KE, Bedford T. Evidence for adaptive evolution in the receptor-binding domain of seasonal coronaviruses OC43 and 229e. eLife 2021;10:e64509 [Full text]
Kitsara I. The patent maze of COVID vaccines and treatments. Presentation at WHO: COVID-19 research and innovation: powering the world’s pandemic response—now and in the future. Day 2, Pt 1. 2022 Feb 24-25 [Presentation at 1:50:00]
Krammer F. Correlates of protection from SARS-CoV-2 infection. Lancet 2021 Apr 17;397(10283):1421-3 [Full text]
Krammer F. Immune responses that confer protection against severe disease and variants, short- and long-term protection. Presentation at WHO R&D blueprint consultation: Developing a framework for evaluating new COVID-19 vaccines. 2022 Feb 23 [Full text]
Krause PR, Arora N, Dowling W, et al. Making more COVID-19 vaccines available to address global needs: considerations and a framework for their evaluation. Vaccine 2022 Sep 22;40(40):5749-51 [Full text]
Kumraj G, Pathak S, Shah S, et al. Capacity building for vaccine manufacturing across developing countries: the way forward. Hum Vaccin Immunother;18(1):2020529 [Full text]
Labombarde JG, Pillai MR, Wehenkel M, et al. Induction of broadly reactive influenza antibodies increases susceptibility to autoimmunity. Cell Rep 2022 Mar 8;38(10):110482 [Full text]
Laidlaw BJ, Ellebedy AH. The germinal centre B cell response to SARS-CoV-2. Nat Rev Immunol 2022 Jan;22(1):7-18 [Full text]
Lancet. Genomic sequencing in pandemics. (Editorial) Lancet 2021 Feb 6;397(10273):445 [Full text]
Lancet Commission on COVID-19 Vaccines and Therapeutics Task Force Members. Operation Warp Speed: implications for global vaccine security. Lancet Glob Health 2021 Jul;9(7):e1017-21 [Full text]
Latinne A, Hu B, Olival KJ, et al. Origin and cross-species transmission of bat coronaviruses in China. Nat Commun 2020 Aug 25;11:4235 [Full text]
Lavelle EC, Ward RW. Mucosal vaccines—fortifying the frontiers. Nat Rev Immunol 2022 Apr;22(4):236-50 [Full text]
Lednicky JA, Tagliamonte MS, White SK, et al. Independent infections of porcine deltacoronavirus among Haitian children. Nature 2021;600(7887):133-7 [Full text]
Lee A, Wimmers F, Pulendran B. Epigenetic adjuvants: durable reprogramming of the innate immune system with adjuvants. Curr Opin Immunol 2022 Aug;77:102189 [Full text]
Letko M, Marzi A, Munster V. Functional assessment of cell entry and receptor usage for SARS-CoV-2 and other lineage B betacoronaviruses. Nat Microbiol 2020 Apr;5(4):562-9 [Full text] (Letko 2020b)
Letko M, Seifert SN, Olival KJ, et al. Bat-borne virus diversity, spillover and emergence. Nat Rev Microbiol 2020;18(8):461-71 [Full text] (Letko 2020a)
Li YD, Chi WY, Su JH, et al. Coronavirus vaccine development: from SARS and MERS to COVID-19. J Biomed Sci 2020;27(1):104 [Full text]
Logue JK, Chu HY. Challenges and lessons in establishing human immune profiling cohort studies for pandemic response. Immunol Rev 2022 Aug;309(1):8-11 [Full text]
Mackin SR, Desai P, Whitener BM, et al. Fcγ receptor-dependent antibody effector functions are required for vaccine protection against infection by antigenic variants of SARS-CoV-2. (Preprint) bioRxiv 2022 Nov 27 [Full text]
MacLean OA, Lytras S, Weaver S, et al. Natural selection in the evolution of SARS-CoV-2 in bats created a generalist virus and highly capable human pathogen. PLOS Biol 2021 Mar;19(3):e3001115 [Full text]
Mani A, Ojha V. Thromboembolism after COVID-19 vaccination: a systematic review of such events in 286 patients. Ann Vasc Surg 2022 Aug;84:12-20.e1 [Full text]
Mannar D, Saville JW, Sun Z, et al. SARS-CoV-2 variants of concern: spike protein mutational analysis and epitope for broad neutralization. Nat Commun 2022 Aug 18;13:4696 [Full text]
Mao T, Israelow B, Peña-Hernández MA, et al. Unadjuvanted intranasal spike vaccine elicits protective mucosal immunity against sarbecoviruses. Science 2022 Nov 25;378(6622):eabo2523 [Full text]
Markov PV, Katzourakis A, Stilianakis NI. Antigenic evolution will lead to new SARS-CoV-2 variants with unpredictable severity. Nat Rev Microbiol 2022;20(5):251-2 [Full text]
Martinez DR, Schäfer A, Leist SR, et al. Chimeric spike mRNA vaccines protect against sarbecovirus challenge in mice. Science 2021 Aug 27;373(6558):991-8 [Full text]
Matuchansky C. Mucosal immunity to SARS-CoV-2: a clinically relevant key to deciphering natural and vaccine-induced defences. Clin Microbiol Infect 2021 Dec;27(12):1724-6 [Full text]
McCray PB Jr, Pewe L, Wohlford-Lenane C, et al. Lethal infection of K18-hACE2 mice infected with severe acute respiratory syndrome coronavirus. J Virol 2007;81(2):813-21 [Full text]
McGrath JJC, Li L, Wilson PC. Memory B cell diversity: insights for optimized vaccine design. Trends Immunol 2022 May;43(5):343-54 [Full text]
McMahan K, Giffin V, Tostanoski LH, et al. Reduced pathogenicity of the SARS-CoV-2 omicron variant in hamsters. (Short communication) Med (N Y) 2022 Apr 8;3(4):262-8.e4 [Full text]
Medicines Patent Pool. MRNA technology transfer hub programme. 2022 [Website]
Meisner J, Baszler TV, Kuehl KE, et al. Household transmission of SARS-CoV-2 from humans to pets, Washington and Idaho, USA. Emerg Infect Dis 2022 Oct 26;28(12):2425-34 [Full text]
Mendelson M, Venter F, Moshabela M, et al. The political theatre of the UK's travel ban on South Africa. (Comment) Lancet 2021 Dec 18;398(10318):2211-3 [Full text]
Mettelman RC, Allen EK, Thomas PG. Mucosal immune responses to infection and vaccination in the respiratory tract. Immunity 2022 May 10;55(5):749-80 [Full text]
Millet JK, Jaimes JA, Whittaker GR. Molecular diversity of coronavirus host cell entry receptors. FEMS Microbiol Rev 2021 May;45(3):fuaa057 [Full text]
Misra A, Theel ES. Immunity to SARS-CoV-2: what do we know and should we be testing for it? J Clin Microbiol 2022 Jun;60(6):e00482-21 [Full text]
Modjarrad K, Moorthy VS, Ben Embarek P, et al. A roadmap for MERS-CoV research and product development: report from a World Health Organization consultation. Nat Med 2016 Jul 7;22(7):701-5 [Full text]
Monrad JT, Sandbrink JB, Cherian NG. Promoting versatile vaccine development for emerging pandemics. NPJ Vaccines 2021 Feb 11;6:26 [Full text]
Morens DM, Taubenberger JK, Fauci AS. Rethinking next-generation vaccines for coronaviruses, influenzaviruses, and other respiratory viruses. Cell Host Microbe 2023 Jan 11;31(1):146-57 [Full text]
Morens DM, Taubenberger JK, Fauci AS. Universal coronavirus vaccines—an urgent need. (Perspective) N Engl J Med 2022 Jan 27;386(4):297-9 [Full text]
Moss P. The T cell immune response against SARS-CoV-2. Nat Immunol 2022 Feb;23(2):186-93 [Full text]
Mostaghimi D, Valdez CN, Larson HT, et al. Prevention of host-to-host transmission by SARS-CoV-2 vaccines. Lancet Infect Dis 2022 Feb;22(2):e52-8 [Full text]
Mudgal R, Nehul S, Tomar S. Prospects for mucosal vaccine: shutting the door on SARS-CoV-2. Hum Vaccin Immunother 2020;16(12):2921-31 [Full text]
Mueni Katee S, Keambou Tiambo C. Discussing the drawbacks of the implementation of access and benefit sharing of the Nagoya Protocol following the COVID-19 pandemic. (Opinion) Front Public Health 2021 Dec 10;9:639581 [Full text]
Munoz FM, Cramer JP, Dekker CL, et al, Brighton Collaboration Vaccine-associated Enhanced Disease Working Group. Vaccine-associated enhanced disease: case definition and guidelines for data collection, analysis, and presentation of immunization safety data. Vaccine 2021 May 21;39(22):3053-66 [Full text]
Muñoz-Fontela C, Dowling WE, Funnell SGP, et al. Animal models for COVID-19. Nature 2020 Oct;586(7830):509-15 [Full text]
Muñoz-Fontela C, Widerspick L, Albrecht RA, et al. Advances and gaps in SARS-CoV-2 infection models. PLOS Pathog 2022 Jan;18(1):e1010161 [Full text]
Nelson SA, Sant AJ. Potentiating lung mucosal immunity through intranasal vaccination. (Perspective) Front Immunol 2021 Dec 14;12:808527 [Full text]
Ng OW, Tan YJ. Understanding bat SARS-like coronaviruses for the preparation of future coronavirus outbreaks—implications for coronavirus vaccine development. Hum Vaccin Immunother 2017 Jan 2;13(1):186-9 [Full text]
Nohynek H. Access and accountability. Presentation at WHO: COVID-19 research and innovation: powering the world’s pandemic response—now and in the future. Day 2, Pt 1. 2022 Feb 24-25 [Presentation at 2:38:00]
Obermeyer F, Jankowiak M, Barkas N, et al. Analysis of 6.4 million SARS-CoV-2 genomes identifies mutations associated with fitness. Science 2022 May 24;376(6599):1327-32 [Full text]
Openshaw PJM. Using correlates to accelerate vaccinology. (Perspective) Science 2022 Jan 6;375(6576):22-3 [Full text]
Oude Munnink BB, Worp N, Nieuwenhuijse DF, et al. The next phase of SARS-CoV-2 surveillance: real-time molecular epidemiology. Nat Med 2021 Sep;27(9):1518-24 [Full text]
Pack SM, Peters PJ. SARS-CoV-2–specific vaccine candidates; the contribution of structural vaccinology. Vaccines 2022;10(2):236 [Full text]
Peacock TP, Penrice-Randal R, Hiscox JA, et al. SARS-CoV-2 one year on: evidence for ongoing viral adaptation. J Gen Virol 2021;102(4):001584 [Full text]
Pecetta S, Kratochvil S, Kato Y, et al. Immunology and technology of severe acute respiratory syndrome coronavirus 2 (SARS-CoV-2) vaccines. Pharmacol Rev 2022 Jan;74(1):313-39 [Full text] (Pecetta 2022b)
Pecetta S, Rappuoli R. mRNA, the beginning of a new influenza vaccine game. Proc Natl Acad Sci USA 2022 Dec 13;119(50):e2217533119 [Full text] (Pecetta 2022a)
Peiris JS, Yuen KY, Osterhaus AD, et al. The severe acute respiratory syndrome. N Engl J Med 2003 Dec 18;349(25):2431-41 [Full text]
Pickering B, Lung O, Maguire F, et al. Highly divergent white-tailed deer SARS-CoV-2 with potential deer-to-human transmission. Nat Microbiol 2022 Dec;7:2011-24 [Full text]
Plotkin SA. Correlates of protection induced by vaccination. Clin Vaccine Immunol 2010 Jul;17(7):1055-65 [Full text]
Plotkin SA. Updates on immunologic correlates of vaccine-induced protection. Vaccine 2020 Feb 24;38(9):2250-7 [Abstract]
Poland GA. Tortoises, hares, and vaccines: a cautionary note for SARS-CoV-2 vaccine development. (Editorial) Vaccine 2020 Jun 2;38(27):4219-20 [Full text]
Poland GA, Ovsyannikova IG, Kennedy RB. The need for broadly protective COVID-19 vaccines: beyond S-only approaches. Vaccine 2021 Jul 13;39(31):4239-41 [Full text]
Prasad SD. Access and accountability. Presentation at WHO: COVID-19 research and innovation: powering the world’s pandemic response—now and in the future. Day 2, Pt 1. 2022 Feb 24-25 [Presentation at 2:15:00]
Qi H, Liu B, Wang X, et al. The humoral response and antibodies against SARS-CoV-2 infection. Nat Immunol 2022 Jun 27;23:1008-20 [Full text]
Qin S, Li R, Zheng Z, et al. Review of selected animal models for respiratory coronavirus infection and its application in drug research. J Med Virol 2022 Jul;94(7):3032-42 [Full text]
Rabalski L, Kosinski M, Mazur-Panasiuk N, et al. Zoonotic spill-over of SARS-CoV-2: mink-adapted virus in humans. (Research notes) Clin Microbiol Infect 2022 Mar;28(3):451.e1-451.e4 [Full text]
Ren W, Qu X, Li W, et al. Difference in receptor usage between severe acute respiratory syndrome (SARS) coronavirus and SARS-like coronavirus of bat origin. J Virol 2008;82(4):1899-1907 [Full text]
Rees H. How can we prospectively evaluate vaccines intended to protect against viruses that are not yet circulating? Presentation at WHO consultation on COVID-19 vaccines research—advancing the development of pan-sarbecovirus vaccines. 2022 Mar 25 [Presentation at 3:17:00] (Rees 2022a)
Rees H. What is the way forward? Presentation at WHO consultation on COVID vaccines research—why do we need a pan-sarbecovirus vaccine? 2022 Jan 28 [Presentation at 5:11:00] (Rees 2022b)
Ribeiro CDS, van Roode MY, Haringhuizen GB, et al. How ownership rights over microorganisms affect infectious disease control and innovation: a root-cause analysis of barriers to data sharing as experienced by key stakeholders. PLOS One 2018 May 2;13(5):e0195885 [Full text]
Rizk JG, Barr CE, Rizk Y, Lewin JC. The next frontier in vaccine safety and VAERS: lessons from COVID-19 and ten recommendations for action. Vaccine 2021 Oct 1;39(41):6017-8 [Full text]
Rizvi Z. Access and accountability. Presentation at WHO: COVID-19 research and innovation: powering the world’s pandemic response—now and in the future. Day 2, Pt 1. 2022 Feb 24-25 [Presentation at 2:31:00]
Rodda LB, Morawski PA, Pruner KB, et al. Imprinted SARS-CoV-2-specific memory lymphocytes define hybrid immunity. Cell 2022 Apr 28;185(9):1588-601.e14 [Full text]
Ruiz-Aravena M, McKee C, Gamble A, et al. Ecology, evolution and spillover of coronaviruses from bats. Nat Rev Microbiol 2022;20(5):299-314 [Full text]
Sampat BN, Shadlen KC. The COVID-19 innovation system. Health Aff (Millwood) 2021 Mar;40(3):400-9 [Abstract]
Sánchez CA, Li H, Phelps KL, et al. A strategy to assess spillover risk of bat SARS-related coronaviruses in Southeast Asia. Nature Commun 2022 Aug 9;13(1):4380 [Full text]
Sauer MM, Tortorici MA, Park YJ, et al. Structural basis for broad coronavirus neutralization. Nat Struct Mol Biol 2021 Jun;28(6):478-86 [Full text]
Saunders KO, Lee E, Parks R, et al. Neutralizing antibody vaccine for pandemic and pre-emergent coronaviruses. Nature 2021 Jun;594(7864):553-9 [Full text]
Seifert SN, Bai S, Fawcett S, et al. An ACE2-dependent sarbecovirus in Russian bats is resistant to SARS-CoV-2 vaccines. PLOS Pathog 2022 Sep;18(9):e1010828 [Full text]
Sekhar A, Kang G. Human challenge trials in vaccine development. Semin Immunol 2020;50:101429 [Full text]
Sette A, Crotty S. Adaptive immunity to SARS-CoV-2 and COVID-19. Cell 2021 Feb 18;184(4):861-80 [Full text]
Sette A, Crotty S. Immunological memory to SARS-CoV-2 infection and COVID-19 vaccines. Immunol Rev 2022 Sep;310(1):27-46 [Full text]
Sharun K, Dhama K, Pawde AM, et al. SARS-CoV-2 in animals: potential for unknown reservoir hosts and public health implications. Vet Q2021;41(1):181-201 [Full text]
Shepherd BO, Chang D, Vasan S, et al. HIV and SARS-CoV-2: tracing a path of vaccine research and development. Curr HIV/AIDS Rep 2022 Feb;19(1):86-93 [Full text]
Sherman AC, Desjardins M, Baden LR. Vaccine-induced severe acute respiratory syndrome coronavirus 2 antibody response and the path to accelerating development (determining a correlate of protection). Clin Lab Med 2022 Mar;42(1):111-28 [Full text]
Sherman AC, Mehta A, Dickert NW, et al. The future of flu: a review of the human challenge model and systems biology for advancement of influenza vaccinology. Front Cell Infect Microbiol 2019 Apr 17;9:107 [Full text]
Shou S, Liu M, Yang Y, et al. Animal models for COVID-19: hamsters, mouse, ferret, mink, tree shrew, and non-human primates. Front Microbiol 2021 Aug 31;12:626553 [Full text]
Shuai H, Chan JF-W, Yuen TT-T, et al. Emerging SARS-CoV-2 variants expand species tropism to murines. eBioMedicine 2021 Nov;73:103643 [Full text]
Siggins MK, Thwaites RS, Openshaw PJM. Durability of immunity to SARS-CoV-2 and other respiratory viruses. Trends Microbiol 2021 Jul;29(7):648-62 [Full text]
Sila T, Sunghan J, Laochareonsuk W, et al. Suspected cat-to-human transmission of SARS-CoV-2, Thailand, July–September 2021. Emerg Infect Dis 2022 Jul;28(7):1485-8 [Full text]
Simon V, Kota V, Bloomquist RF, et al. PARIS and SPARTA: finding the Achilles' heel of SARS-CoV-2. mSphere 2022 May-Jun;7(3):e00179-22 [Full text]
Simons LM, Ozer EA, Gambut S, et al. De novo emergence of SARS-CoV-2 spike mutations in immunosuppressed patients. Transpl Infect Dis 2022 Dec;24(6):e13914 [Full text]
Singh A, Singh RS, Sarma P, et al. A comprehensive review of animal models for coronaviruses: SARS-CoV-2, SARS-CoV, and MERS-CoV. Virol Sin 2020 Jun;35(3):290-304 [Full text]
Singh J, Pandit P, McArthur AG, et al. Evolutionary trajectory of SARS-CoV-2 and emerging variants. Virol J 2021;18:166 [Full text]
Soraci L, Lattanzio F, Soraci G, et al. COVID-19 vaccines: current and future perspectives. Vaccines (Basel) 2022 Apr 13;10(4):608 [Full text]
Starr TN, Czudnochowski N, Liu Z, et al. SARS-CoV-2 RBD antibodies that maximize breadth and resistance to escape. Nature 2021 Sep 2;597(7874):97-102 [Full text]
Starr TN, Zepeda SK, Walls AC, et al. ACE2 binding is an ancestral and evolvable trait of sarbecoviruses. Nature 2022 Mar;603(7903):913-8 [Full text]
Subbarao K. The success of SARS-CoV-2 vaccines and challenges ahead. Cell Host Microbe 2021 Jul 14;29(7):1111-23 [Full text]
Sui Y, Bekele Y, Berzofsky JA. Potential SARS-CoV-2 immune correlates of protection in infection and vaccine immunization. Pathogens 2021 Feb;10(2):138 [Full text]
Sun J, Zhuang Z, Zheng J, et al. Generation of a broadly useful model for COVID-19 pathogenesis, vaccination, and treatment. Cell 2020 Aug 6;182(3):734-43.e5. [Full text]
Sung HD, Kim N, Lee Y, et al. Protein-based nanoparticle vaccines for SARS-CoV-2. Int J Mol Sci 2021;22(24):13445 [Full text]
Suryawanshi R, Ott M. SARS-CoV-2 hybrid immunity: silver bullet or silver lining? Nat Rev Immunol 2022 Oct;22(10):591-2 [Full text]
Tan CCS, Lam SD, Richard D, et al. Transmission of SARS-CoV-2 from humans to animals and potential host adaptation. Nat Commun 2022 May 27;13:2988 [Full text]
Tan CW, Chia WN, Young BE, et al. Pan-sarbecovirus neutralizing antibodies in BNT162b2-immunized SARS-CoV-1 survivors. (Brief report) N Engl J Med 2021 Oct 7;385(15):1401-6 [Full text]
Tarke A, Coelho CH, Zhang Z, et al. SARS-CoV-2 vaccination induces immunological T cell memory able to cross-recognize variants from Alpha to Omicron. Cell 2022 Mar 3;185(5):847-59.e11 [Full text]
Telenti A, Hodcroft EB, Robertson DL. The evolution and biology of SARS-CoV-2 variants. Cold Spring Harb Perspect Med 2022 May 27;12(5):a041390 [Full text]
Temmam S, Vongphayloth K, Baquero E, et al. Bat coronaviruses related to SARS-CoV-2 and infectious for human cells. Nature 2022 Apr 14;604(7905):330-6 [Full text]
Terrier O, Si-Tahar M, Ducatez M, et al. Influenza viruses and coronaviruses: knowns, unknowns, and common research challenges. PLOS Pathog 2021 Dec;17(12):e1010106 [Full text]
Tomalka JA, Suthar MS, Deeks SG, et al. Fighting the SARS-CoV-2 pandemic requires a global approach to understanding the heterogeneity of vaccine responses. Nat Immunol 2022 Mar;23(3):360-70 [Full text]
Trichel AM. Overview of nonhuman primate models of SARS-CoV-2. Comp Med 2021 Oct;71(5):411-32 [Full text]
University of Oxford. COV-CHIM01: SARS-CoV-2 (COVID-19) dose finding infection study. ClinicalTrials.gov Identifier: NCT04864548. First posted 2021 Apr 29; updated 2023 Jan 6 [Trial record]
US DDHS (Department of Health and Human Services). Framework for guiding funding decisions about proposed research involving enhanced potential pandemic pathogens. 2017:1-6 [Full text]
US FDA (Food and Drug Administration). Animal rule information. Accessed 2022 Dec 21 [Web page]
US FDA. FDA approves first COVID 19 vaccine. 2021 Aug 23 [News release]
US FDA. Guidance for Industry: General principles for the development of vaccines to protect against global infectious diseases. Dec 2011 [Full text]
van Kampen JJA, van de Vijver DAMC, Fraaij PLA, et al. Duration and key determinants of infectious virus shedding in hospitalized patients with coronavirus disease–2019 (COVID-19). Nat Commun 2021 Jan 11;12:267 [Full text]
Vardhana S, Baldo L, Morice WG 2nd, et al. Understanding T-cell responses to COVID-19 is essential for informing public health strategies. (Viewpoint) Sci Immunol 2022 Mar 24;7(71):eabo1303 [Full text]
Verbeke R, Hogan MJ, Loré K, et al. Innate immune mechanisms of mRNA vaccines. Immunity 2022 Nov 8;55(11):1993-2005 [Full text]
Wahl I, Wardemann H. Sterilizing immunity: understanding COVID-19. Immunity 2022 Dec 13;55(12):2231-5 [Full text]
Walls AC, Miranda MC, Schäfer A, et al. Elicitation of broadly protective sarbecovirus immunity by receptor-binding domain nanoparticle vaccines. Cell 2021 Oct 14;184(21):5432-47.e16 [Full text]
Wang M, Yan M, Xu H, et al. SARS-CoV infection in a restaurant from palm civet. Emerg Infect Dis 2005;11(12):1860-5 [Full text]
Wang S, Li L, Yan F, et al. COVID-19 animal models and vaccines: current landscape and future prospects. Vaccines (Basel) 2021 Oct;9(10):1082 [Full text]
Wells HL, Letko M, Lasso G, et al. The evolutionary history of ACE2 usage within the coronavirus subgenus sarbecovirus. Virus Evol 2021 Jan;7(1):veab007 [Full text]
Wherry EJ, Barouch DH. T cell immunity to COVID-19 vaccines. (Perspective) Science 2022 Aug 19;377(6608):821-2 [Full text]
WHO (World Health Organization). Coronavirus (COVID 19) dashboard. Accessed 2022 Dec 20 [Web page] (WHO 2022a)
WHO. COVID-19 vaccine tracker and landscape. 2023 (updates semiweekly) [Web page]
WHO. C-TAP: a concept paper. 2020 Oct 27 [Full text] (WHO 2020a)
WHO. Global Influenza Surveillance and Response System (GISRS). Accessed 2022 Oct 3 [Web page] (WHO 2022b)
WHO. Implementation of the Nagoya Protocol and pathogen sharing: public health implications. 2017 Feb 1 [Full text]
WHO. Moving forward on goal to boost local pharmaceutical production, WHO establishes global biomanufacturing training hub in Republic of Korea. 2022 Feb 23 [News release] (WHO 2022c)
WHO. Q&A for guidelines on emergency use listing procedure. 2020 Jul 15 [Full text] (WHO 2020b)
WHO. R&D Blueprint. Accessed 2022 Nov 21 [Web page]
WHO. The mRNA technology transfer hub. Accessed 2022 Jul 5 [Web page] (WHO 2022d)
WHO. WHO global benchmarking tool (GBT) for evaluation of national regulatory system of medical products—Revision VI. 2021 May 10 [Full text]
WHO IVB (Immunizations, Vaccines, and Biologics) Product and Delivery Research unit. Preferred product characteristics and target product profiles. Accessed 2023 Jan 12 [Web page]
WIPO (World Intellectual Property Organization). COVID-19-related vaccines and therapeutics: preliminary insights on related patenting activity during the pandemic. 2022 [Full text]
Williams E, Craig K, Chiu C, et al. Ethics review of COVID-19 human challenge studies: a joint HRA/WHO workshop. Vaccine 2022 Jun 9;40(26):3484-9 [Full text]
Witt AN, Green RD, Winterborn AN. A meta-analysis of rhesus macaques (Macaca mulatta), cynomolgus macaques (Macaca fascicularis), African green monkeys (Chlorocebus aethiops), and ferrets (Mustela putorius furo) as large animal models for COVID-19. Comp Med 2021 Oct;71(5):433-41 [Full text]
Wong LR, Zheng J, Wilhelmsen K, et al. Eicosanoid signalling blockade protects middle-aged mice from severe COVID-19. Nature 2022 May 5;605(7908):146-51 [Full text]
Woo PC, Lau SK, Huang Y, et al. Coronavirus diversity, phylogeny and interspecies jumping. Exp Biol Med (Maywood) 2009 Oct;234(10):1117-27 [Full text]
Worobey M, Levy JI, Serrano LM, et al. The Huanan Seafood Wholesale Market in Wuhan was the early epicenter of the COVID-19 pandemic. Science 2022 Aug 26;377(6609):951-9 [Full text]
Wuertz KM, Barkei EK, Chen WH, et al. A SARS-CoV-2 spike ferritin nanoparticle vaccine protects hamsters against Alpha and Beta virus variant challenge. NPJ Vaccines 2021 Oct 28;6:129 [Full text]
Xiong Q, Cao L, Ma C, et al. Close relatives of MERS-CoV in bats use ACE2 as their functional receptors. Nature 2022 Dec 22;612(7941):748-57 [Full text]
Yewdell JW. Antigenic drift: understanding COVID-19. Immunity 2021 Dec 14;54(12):2681-7 [Full text] (Yewdell 2021a)
Yewdell JW. Individuals cannot rely on COVID-19 herd immunity: durable immunity to viral disease is limited to viruses with obligate viremic spread. PLOS Pathog 2021 Apr 26;17(4):e1009509 [Full text] (Yewdell 2021b)
Yu D, Walker LSK, Liu Z, et al. Targeting TFH cells in human diseases and vaccination: rationale and practice. Nat Immunol 2022 Aug;23(8):1157-68 [Full text]
Yu ED, Narowski TM, Wang E, et al. Immunological memory to common cold coronaviruses assessed longitudinally over a three-year period pre-COVID19 pandemic. Cell Host Microbe 2022 Sep 14;30(9):1269-78.e4 [Full text]
Yuan M, Wu NC, Zhu X, et al. A highly conserved cryptic epitope in the receptor binding domains of SARS-CoV-2 and SARS-CoV. Science 2020;368(6491):630-3 [Full text]
Zaki AM, van Boheemen S, Bestebroer TM, et al. Isolation of a novel coronavirus from a man with pneumonia in Saudi Arabia. (Brief report) N Engl J Med 2012 Nov 8;367(19):1814-20 [Full text]
Zarnitsyna VI, Ellebedy AH, Davis C, et al. Masking of antigenic epitopes by antibodies shapes the humoral immune response to influenza. Philos Trans R Soc Lond B Biol Sci 2015 Sep 5;370(1676):20140248 [Full text]
Zheng MZM, Wakim LM. Tissue resident memory T cells in the respiratory tract. Mucosal Immunol 2022 May;15(3):379-88 [Full text]
Zehr JD, Pond SLK, Martin DP, et al. Recent zoonotic spillover and tropism shift of a canine coronavirus is associated with relaxed selection and putative loss of function in NTD subdomain of spike protein. Viruses 2022 Apr 21;14(5):853 [Full text]
Zhou P, Song G, He WT, et al. Broadly neutralizing anti-S2 antibodies protect against all three human betacoronaviruses that cause severe disease. (Preprint) bioRxiv 2022 Mar 7 [Full text]
Zhou Z, Ali A, Walelign E, et al. Genetic diversity and molecular epidemiology of Middle East Respiratory Syndrome Coronavirus in dromedaries in Ethiopia, 2017 to 2020. Emerg Microbes Infect 2023 Jan 9:2164218 [Full text]
Zhou Z, Hui KPY, So RTY, et al. Phenotypic and genetic characterization of MERS coronaviruses from Africa to understand their zoonotic potential. Proc Natl Acad Sci USA 2021 Jun 22;118(25):e2103984118 [Full text]
Zhu N, Zhang D, Wang W, et al. A novel coronavirus from patients with pneumonia in China, 2019. N Engl J Med 2020 Feb 20;382(8):727-33 [Full text]
Ziogas A, Netea MG. Trained immunity-related vaccines: innate immune memory and heterologous protection against infections. Trends Mol Med 2022 Jun;28(6):497-512 [Abstract]
Zmasek CM, Lefkowitz EJ, Niewiadomska A, et al. Genomic evolution of the Coronaviridae family. Virology 2022 May;570:123-33 [Full text]
ABSL Animal biosafety level
ACE2 Angiotensin converting enzyme-2
BSL Biosafety level
C-TAP COVID-19 Technology Access Pool
CEPI Coalition for Epidemic Preparedness Innovations
CFR Case-fatality ratio
CHIM Controlled human infection model
CIDRAP Center for Infectious Disease Research and Policy (University of Minnesota)
CoP Correlate of protection
COVAX COVID-19 Vaccines Global Access
COVID-19 Coronavirus Disease 2019
CVR Coronavirus Vaccines R&D Roadmap
DNA Deoxyribonucleic acid
DPP4 Dipeptidyl peptidase 4
FcR Fc receptor
FVVA Full value of vaccine assessment
GCP Good clinical practice
GISAID Global Initiative on Sharing All Influenza Data
GISRS Global Influenza Surveillance and Response System
GLP Good laboratory practice
GMP Good manufacturing practice
hACE2 Human angiotensin converting enzyme-2
HICs High-income countries
IgA Immunoglobulin A
IgG Immunoglobulin G
LMICs Low- and middle-income countries
ME&A Monitoring, evaluation, and adjustment
MERS-CoV Middle East respiratory syndrome coronavirus
mRNA Messenger ribonucleic acid
MICs Middle-income countries
NHP Nonhuman primate
NIAID National Institute of Allergy and Infectious Diseases
NIH National Institutes of Health
NRA National regulatory authority
NTD N-terminal domain
PASC Post-acute sequelae of SARS-CoV-2 infection
PPCs Preferred product characteristics
R&D Research and development
RBD Receptor binding domain
RCT Randomized controlled trial
RNA Ribonucleic acid
S Spike (spike protein)
SARS-CoV Severe acute respiratory syndrome coronavirus
SARS-CoV-2 Severe acute respiratory syndrome coronavirus 2
SMART Specific, measureable, achievable, realistic/relevant, and time-sensitive
SME Subject-matter expert
Th T helper (T helper cells)
TPP Target product profile
TRIPS Trade Related Aspects of Intellectual Property Rights
TRM Tissue resident memory
US FDA United States Food and Drug Administration
VAED Vaccine-associated enhancement of disease
VOCs Variants of concern
WHO World Health Organization
WTO World Trade Organization
For the list of steering group members, taskforce members, CIDRAP contributing authors, and CIDRAP editorial/design staff who contributed to the development of the R&D Roadmap for Broadly Protective Coronavirus Vaccines, see Appendix B in the PDF or the CVR website About page.
Topic 1: Virology Applicable to Vaccine R&D |
Strategic Goal 1.1: Enhance and sustain the capacity to identify, characterize, and share SARS-CoV-2 variants of interest, concern, and high consequence among researchers globally. |
Milestone 1.1.d: By 2024, generate a financially sustainable, collaborative international program to quickly identify, characterize, and share information on SARS-CoV-2 viruses, including antigenic information, in real time with the potential to build on current systems such as the WHO’s Global Influenza Surveillance and Response System (GISRS) (WHO 2022b, Harvey 2021, Subbarao 2021). |
Strategic Goal 1.2: Improve characterization of the coronavirus universe to determine the diversity of strains necessary to ensure adequate breadth of coverage for vaccine R&D. |
Milestone 1.2.c: By 2024, devise a consensus approach to prioritize and select coronaviruses that would constitute an optimally diverse panel to be used in vaccine R&D for assessing breadth of protection (Baric 2022). Selection criteria should initially focus on alphacoronaviruses and betacoronaviruses that: (1) use the hACE2 receptor, (2) grow in primary human cells, (3) are genetically diverse, (4) have been antigenically characterized, and (5) have strains available for study. |
Milestone 1.2.f: By 2024, generate at least one initial panel of virus stocks featuring different coronaviruses and diverse cell lines that are readily susceptible to a wide range of coronaviruses, and make the panel accessible to researchers working on coronavirus vaccine R&D (Letko 2020a, Ruiz-Aravena 2022). |
Topic 2: Immunology and Immune Correlates of Protection |
Strategic Goal 2.1: Ensure that clinical samples and immunoassays are available to the research community for improving understanding of the mechanisms of mucosal and systemic immunity related to SARS-CoV-2 infection. |
Milestone 2.1.a: By 2023, develop a centralized or virtual biorepository, and an associated governance structure, to use pre-COVID-19 pandemic clinical samples, including mucosal (e.g., nasal lavage and saliva) and serologic samples that are currently available from a range of research laboratories, potentially by tapping into existing biobanks. Milestone 2.1.c: By 2024, establish and fund a centralized or virtual biorepository involving a new cohort of subjects from multiple regions of the world, to include those with a history of SARS-CoV-2 infection, for obtaining high-impact (e.g., mucosal, bronchoalveolar lavage, serologic, bone marrow) and appropriately collected and timed clinical samples. Milestone 2.1.d: By 2024, develop new immunologic assays for SARS-CoV-2 research, as outlined in the plan identified in Milestone 2.1.b, and ensure that such assays are appropriately harmonized, standardized, and reproducible. |
Strategic Goal 2.2: Define mechanisms of mucosal and systemic immunity relevant to SARS-CoV-2 infection and the development of broadly protective coronavirus vaccines. |
Milestone 2.2.d: By 2027, determine mucosal biomarkers, including systemic surrogates of mucosal immunity (Matuchansky 2021), that are predictive of mucosal immune protection against SARS-CoV-2 infection. |
Strategic Goal 2.3: Clarify mechanisms for stimulating broadly protective mucosal and systemic immune responses that are cross-reactive for different coronaviruses. |
Milestone 2.3.a: By 2024, identify epitopes other than the RBD area of the S protein that generate protective humoral immunity and are conserved across virus types (Cohen J 2021, Crowe 2022, Martinez 2021, Saunders 2021, Walls 2021). |
Strategic Goal 2.4: Understand the mechanisms of durability of immune protection from SARS-CoV-2 and other coronaviruses. |
Milestone 2.4.a: By 2024, determine initial factors that influence duration of antibody and memory B- and T-cell responses following SARS-CoV-2 infection or vaccination, such as persistence of the germinal center (Bhattacharya 2022, Moss 2022, Siggins 2021, Tarke 2022). |
Strategic Goal 2.6: Identify mechanistic correlates of protection for immunity generated by SARS-CoV-2 vaccines and broadly protective coronavirus vaccines. |
Milestone 2.6.c: By 2026, identify statistically validated CoPs for predicting the efficacy of SARS-CoV-2 vaccines based on key immune responses that correlate with specific clinical end points and that are applicable to viral variants with different characteristics. Milestone 2.6.d: By 2027, determine one or more CoPs for mucosal vaccines against SARS-CoV-2 infection. |
Topic 3: Vaccinology |
Strategic Goal 3.1: Define goals for broadly protective coronavirus vaccines by establishing a widely agreed upon and vetted set of PPCs and determine use cases for such vaccines. |
Milestone 3.1.a: By 2023, building on existing TPPs, develop a broadly agreed upon and internationally vetted (e.g., through a process involving an international multilateral organization such as the WHO) set of PPCs to identify key product characteristics, including optimal and critical minimal criteria. These could follow a tiered approach, with an initial focus on variant-proof SARS-CoV-2 vaccines, then moving to other, more broadly protective tiers. |
Strategic Goal 3.2. Leverage new technologies or new approaches to create effective, durable, and safe vaccines that offer broad protection across different coronaviruses. |
Milestone 3.2.b: By 2023, publish the findings of a workshop on SARS-CoV-2 mucosal vaccines to identify gaps in mucosal approaches for vaccine development. Milestone 3.2.d: By 2024, develop and make available to researchers an initial repository of coronaviruses (as available), pseudoviruses (if they can be made), and antigens. The repository could be developed in a tiered fashion, with an initial focus on the highest-risk viruses and then adding additional viruses over time. Milestone 3.2.i: By 2027, further clarify, through clinical studies, if alternative routes of administration, including intranasal, transdermal, inhaled, and oral vaccines, can enhance mucosal immunity and protect against disease and transmission. |
Strategic Goal 3.4. Build a foundation for regulatory evaluation of future coronavirus vaccines. |
Milestone 3.4.b: By 2023, develop a set of principles to inform regulatory evaluation of new coronavirus vaccines that outlines what information is required to demonstrate the need for and added value of variant-proof SARS-CoV-2 vaccines and to provide confidence in vaccine efficacy, particularly compared to approved vaccines. This set of principles would be less specific than regulatory guidance, since many details will still be unresolved, but such principles could be a valuable starting point for clarifying regulatory evaluation for such vaccines. Milestone 3.4.c: By 2025 and building on Milestone 3.4.b, develop a set of principles for regulatory evaluation of future more broadly protective coronavirus vaccines that: (1) follows a tiered or stepwise approach (such as starting with predicting efficacy against sarbecoviruses other than SARS-CoV-2, then to merbecoviruses, and then to additional coronaviruses of concern as necessary); (2) takes into consideration the various mechanisms of protection that different vaccines may employ, which may inform the potential breadth of protection for a given vaccine construct; (3) clarifies what a “broadly protective coronavirus vaccine” means from a regulatory perspective and how breadth of protection is communicated in the associated product information; (4) identifies approaches for predicting protection provided by new vaccines (i.e., predicting potential clinical benefit) against coronaviruses that are not circulating in the human population; (5) defines the potential roles and limitations of tools such as animal studies, human infection studies, and immunobridging for predicting the breadth of protection for new vaccines; and (6) clarifies potential regulatory pathways for new coronavirus vaccines. |
Strategic Goal 3.5. Facilitate the development of vaccine candidates with characteristics that meet global needs. |
Milestone 3.5.c: By 2027, support the development of coronavirus vaccine technologies that are suitable for broad access and global distribution, such as cold-chain–independent technologies; are scalable; and can be produced affordably. |
Topic 4: Animal and Human Infection Models for Coronavirus Vaccine Research |
Strategic Goal 4.1: Ensure that appropriate animal models are developed and available for conducting R&D for broadly protective coronavirus vaccines. |
Milestone 4.1.a: By 2024, develop a strategy to ensure that validated, reliable reagents; virus strains and stocks; and harmonized serologic assays are available for studying a broader range of coronaviruses in animal models, with an initial focus on additional sarbecoviruses (group 2b betacoronaviruses) and a wider variety of MERS-related merbecoviruses (group 2c betacoronaviruses). Milestone 4.1.b: By 2024, convene an international workshop on animal models for studying broadly protective coronavirus vaccines. Examples of topics for the workshop include: (1) review existing animal models for SARS-CoV, SARS-CoV-2, MERS-CoV, and other coronaviruses; (2) determine which animal models are best suited for R&D of broadly protective coronavirus vaccines; (3) identify strategies to optimize the use of small-animal models (e.g., mice, hamsters, ferrets); (4) determine how best to optimize and reduce the use of NHPs for R&D efforts, particularly given their limited supply; (5) determine how to mimic preexisting immunity in animal models; (6) determine how animal models can be used to assess the impact of host genomics or the microbiome on vaccine performance, such as through the use of “dirty mice”; (7) determine the role of animal models in measuring mucosal immunity, breadth, and durability of vaccines; (8) determine the role of animal models in defining immune CoPs; (9) determine the role of animal models in studying long COVID/PASC; (10) identify gaps in the current animal model landscape; and (11) develop strategies and plans for meeting future animal-model research needs. Milestone 4.1.d: By 2025, ensure that standardized, validated, and well-characterized animal models are available to evaluate and compare broadly protective coronavirus vaccines. Examples of parameters to consider include the challenge virus strain; dose, route, volume, and timing of challenge; and animal responses to human-adapted variants. Immune history and prior exposure to ancestral coronaviruses should also be considered. The appropriate surrogate markers of clinical disease severity, such as weight loss or virus titers in the lungs, are needed for each animal species and for each virus sub-genus or relevant variant used to establish the model. |
Strategic Goal 4.2: Establish the role of a CHIM in R&D for broadly protective coronavirus vaccines and optimize the model for vaccine research. |
Milestone 4.2.c: By 2024, develop a set of best practices for using a CHIM in coronavirus vaccine research to include risk mitigation strategies that reflect the changing landscape of disease and therapies. Milestone 4.2.d: By 2025, establish parameters, in coordination with global regulators, for using CHIM studies and immunobridging for licensure of candidate vaccines. Milestone 4.2.e: By 2025—assuming candidate vaccines are available—standardize parameters for a CHIM model in assessing broadly protective coronavirus vaccines, such as determining appropriate strain selection (which may need to be defined contextually at the time), standardizing panels of immunologic assays and assay harmonization, identifying mucosal inflammatory markers, and harmonizing protocols as possible. |
Topic 5: Policy and Financing |
Strategic Goal 5.1. Establish and convey the value of sustained financial support and demand for developing broadly protective coronavirus vaccines. |
Milestone 5.1.a: By 2024, develop and disseminate a detailed economic case for broadly protective coronavirus vaccines through a full value of vaccine assessment (FVVA) or a series of detailed cost-benefit analyses for vaccines from SARS-CoV-2 variant-proof vaccines to more broadly protective coronavirus vaccines. These assessments will need to include a multitude of perspectives (e.g., health payers, economic, and societal) at a number of levels (e.g., global, national, and regional) and take into account varying contexts (e.g., demographics, healthcare capacity, competing health priorities) and the potential pathways for deployment, such as routine, preventive use or reactive outbreak control (Giersing 2021). Milestone 5.1.c: By 2024, convene a meeting of vaccine investors, purchasers (including governments and large global institutions), producers, and governmental representatives aimed at exploring strategies for providing a reliable marketplace and financial model for broadly protective coronavirus vaccines. Meeting participants will assess the current push (e.g., grants, subsidies) and pull incentives (e.g., advance market commitments) and appropriate thresholds to move from push to pull, as well as establish a pricing model in line with the PPCs that can be anticipated for vaccines of various characteristics, such as the number of doses required, stability, duration of protection, and level of protection. |